1. Introduction
Landsliding is the dominant mass-wasting process in the mountainous area (Burbank et al., 1996; Hovius et al., 2000; Meunier et al., 2008; Brunetti et al., 2014), where young streams continuously undercut the slopes. A significant challenge in landslide investigation is to identify the slope failure mechanism under different physiography and its relationship with slope material compositions. A critical relationship was found between the characteristics of slope materials and the composition of clay-silt sediment on the sliding surface; and the slope failure mechanism (Summa et al., 2010; Azañón et al., 2010; Brunet et al., 2016; Summa et al., 2018). Geomorphological investigation of slope instability is very crucial to understanding the causes of a landslide in geo-dynamically active terrain like the Garhwal Himalaya. The factors which induce landslides in the area include; seismotectonic movements (Brunsden et al., 1981; Owen, 1991; Owen et al., 1995, 1996; Owen and Sharma, 1998; Barnard et al., 2001), heavy and prolonged spells of rainfall leading to saturation and increasing the incumbent load on the slope that substantially increase shear stress (Bartarya and Sah, 1995; Paul et al., 2000; Srivastava et al., 2013; Owen et al., 1996; Shroder and Bishop, 1998); and anthropogenic activity (Barnard et al., 2001; Begueria 2006; Glade, 2003; Remondo et al., 2005).
The reduction of shear strength is the principal cause of slope instability and often triggers landslides. Therefore, it is essential to analyze the material characteristics of the landslide slip zone to understand the fundamental mechanisms of slope instability, and to delineate susceptible areas (Baoping and Haiyang, 2007). Hence, analysis of the sedimentary depositional environment can provide crucial insight into the displacement mechanism of materials (Dufresne et al., 2016; Xiaomin et al., 2019). Shear strength reduction at the slope slip zone could be a result of mineral composition, grain size, and the processes that are operating over the slope. Therefore, a comprehensive investigation of slope material is required to analyse the mineral composition, lithology, and grain morphology, under which landslide occurs (Wiemer et al., 2015).
The mineral and chemical composition of a landslide-slip zone material, generally those of slide bodies and bedrocks, could provide insight into the landslide characteristics. It is considered to be one of the effective ways to examine the causes of instability in the landslide-slip zone, and the mechanism of slope failures (Dill, 1998). Although, the x-ray diffraction analysis of the mineralogical composition of slope material does not have a direct relationship with landslide yet; it was used in another way, such as site-specific sediment mineralogy for understanding the mechanism of landslide slip zone (Cafaro and Cotecchia, 2001; Bogaard et al., 2007; Summa et al., 2010; Xie et al., 2022). The abundance of Fe2O3 minerals within the slip zone of landslide materials is saturated due to rain-water infiltration and groundwater, leading to the hydrolysis of quartz, and the formation of clay minerals, particularly kaolinite (Summa et al., 2010). Hence, the increase of clay minerals concentration in the slope material, particularly at the slip-zone, acts as a lubricant and reduces shear strength; and activates landslide (Summa et al., 2010; Wang and Sassa, 2003).
Ascertaining links between slope failure mechanism and micro-surface features present on the slope materials can provide insight into slope failure. Systematic studies of quartz grains offer comprehensive information on sedimentary histories such as erosion, deposition, and the tectonic environment (Krinsley and Doornkamp, 1973; Mahaney et al., 2001). The presence of micro-surface texture digenetic changes can be analyzed to understand the alteration, and cementation of original sediments, and the nature of pore-fluid that moved through the sedimentary sequence (Tucker, 1988; Mahaney, 2002; Moral Cardona et al., 2005; Pandey, 2011). Sedimentological properties have been found to reflect the interaction between source material, energy, and climatic regimes in high mountainous areas (Sharma, 1996). Therefore, particle size, micro-fabric, and quartz grain texture analysis are very significant for the identification of processes and intensity of slope instability (Pandey, 2011; Vos et al., 2014). The term slope failures, which includes all types of the mass movement, has been used as a synonym for landslide in the present study. To understand the surface processes; that cause slope instability, sediment samples were collected from landslides and processed using Sieve analysis, X-ray Diffraction (XRD) powder, and Scanning Electron Microscopy (SEM) techniques.
2. Geological setting of the area
The Bhilangana basin covers both the Lesser and Greater Himalayan ranges, separated by the Main Central Thrust (MCT). The area is seismically very active, and a large number of earthquakes occur along MCT ((https://earthquake.usgs.gov/earthquakes/map/?), inferring continual neo-tectonic activity along the thrust plane (Fig. 1). Lithological formations of the area include the Higher Himalayan Crystalline (HHC) that formed of granite and gneiss of Vakirta and Munisiari groups to the north of MCT. The Lesser Himalayan rock group includes two types of formations, i.e., the Lesser Himalayan Crystalline (LHC) of Ramgarh group and the Lesser Himalayan Sedimentaries (LHS) including Subathu, Tejam, Damtha and Chandpur formations (Valdiya, 1980; Pandey, 2011).
The geotectonic setup and lithology of the Garhwal Himalaya represent Quaternary to Protozoic formations (Fig. 1) with three distinct geotectonic units. Two of which are located in the Central Himalayan Crystalline Group of rocks; and the third is Deoban/Garhwal tectonic unit. The crystalline and sedimentary rocks are separated from each other by Thayeli thrust (Rao and Pati, 1982; Valdiya, 1980). It is a low-angle thrust separating these two tectonic units and is given the site-specific name of Thayeli. A major north-south direction fault, known as the Balganga fault, offsets the Thayeli thrust from a distance of 50 km in the Balganga valley (Sakliani, 1989).
The Central Himalayan Crystalline rocks are subdivided into two distinct geological units separated from each other by a tectonic plane (Table 1). The lower Bhilangana Formation comprises low to medium-grade metamorphoses such as porphyroblastic biotite streaky genesis, quartzite, quartz-schist, quartz-felspathic schist, chlorite schist persistent limestone bands, epidiorite, and amphibolite. Medium represents the higher unit, the Gangi-Kedarnath formation to high-grade metamorphics like garnetiferous quartz schist, biotite schist, graphite schist, carbonaceous phyllite, porphyroblastic gneiss, granulites, marble, and amphibolite. The upper Proterozoic rocks include Bhilangana, Ghansali formation, and Garhwal/Deoban basic volcanic rocks. The Proterozoic rock groups constitute gneisses, migmatites, and intercalated metasediments known as the Vakirta group (Naqvi, 2005).
Table 1 Tectonic Sequence and lithological unit in Bhilangana Basin (modified after; Rao and Pati, 1980).
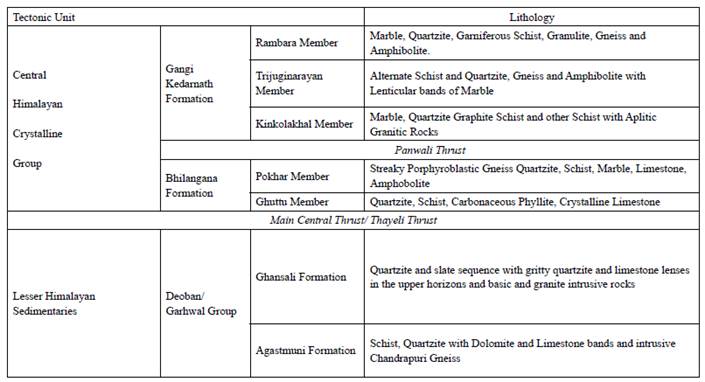
3. Materials and methods
During the fieldwork of September 2009, we surveyed 135 landsides, out of which 25 sites were selected for the sedimentological analysis to understand the failure mechanism (Table 2). Sediment samples collected from each landslide occurred in the different lithological formations and were well distributed in the study area (Fig. 2). As sediment samples were collected from the failed slope materials, it necessitates collecting the mixture of material across the landslide body to understand its instability process. The samples were taken from three positions for each site including slide head, intact materials, and dislodged material. In addition to the measurement of landslide geometry, in-situ rock strength was also assessed using the PROCEQ DIGI-SCHMIDT 2000 (Concrete Test Hammer Model ND/LD); also termed an original hammer. Schmidt hammer uses the energy of impact released by a spring-controlled plunger. We have taken ten impacts point in each slope material across the slide body, and the mean value of these measurements was used as rock mass strength (Mpa).
Grain-size distribution analyses of sediment were carried out using a motorized sieve shaker with sieve mesh sizes varying from 63 urn to 2 mm, capable of differentiating grain size intervals from 0.2 to 200 urn. Sediment samples collected from the sites typically contain moisture, and therefore, are air-dried at room temperature for three weeks. On average, 500 gm of the sample was used for grain size distribution. Since these samples have coarse grains, the optimum weight of 500-1000 grams of sediment is usually preferred for this analysis. The duration of the mechanical shaking of samples was kept 15 minutes for each sample. The standard time used for processing in most laboratories is 15-20 minutes but it is recommended to be 10-15 minutes for samples consisting of coarse grains (Pye, 2007).
The mineralogical analysis was carried out by X-ray diffraction (XRD), using Pan Analytical PW3050 XPERT-PRO powder diffractometer (CuKa radiation and secondary monochromatic sample spinner). The procedure was applied to obtain mineralogical data of bulk rock using the Barahona Fernandez (1974) method. This technique depends on the integrated intensities of measurements of all the crystalline phases. However, the results obtained were cross-checked, comparing mineralogical library data. The scan axis position (°2Th) was taken to be 5.03 to 89.99 with continuous scan type and logging interval of one second. The sediment size was < 2µm crystal powdered mounted in a substantial sample holder. The analyses of mixed-layer clay properties were performed using Moore and Reynolds (1989) and semi-quantitative estimates succeeding Biscaye (1965); with small amendments.
Further, SEM analysis and powder diffraction (XRD) techniques were used in this study to analyze the crystallographic structure, crystallite size (grain size), and preferred orientation in polycrystalline powdered bedrock sediments. Diffraction peaks of unknown substances were compared with the diffraction database maintained by the International Centre for Diffraction Data to identify the mineralogical composition present in the sediments. Also, powder diffraction is a standard method used for determining lattice strains in crystalline materials.
SEM analyses of quartz grains have been done to identify the microsurface texture, morphological features, and processes. The quartz grains were separated from sediment samples and dipped for 20 minutes in hydrochloric acid (HCL) to remove impurities. Though quartz grains are a highly conductive element, the gold coating (Au) of these grains made for better conductivity. Carl Zeiss EVO 40 instrument, on 20 kV was used for SEM analysis. Quartz sand grains were mounted on 10mm diameter aluminium stubs using double-sided carbon adhesive discs. It was considered sufficient to photomicrograph and measurement of grains into two orthogonal orientations. The grains are mounted in a linear array, as on the left-hand stub. The measurements and photomicrographs were acquired into three orientations of grains mounted on a triangular array to study elongation, flatness, and sphericity.
The proposition is that surface textural features and assemblage records provide information about the origin, transport history, and subsequent weathering processes or diagenetic processes of materials investigated. Presence or absence of different types of features recorded, either regarding presence/ absence or relative abundance; such as absent or rare (<5% of grains), scant (5 to 25%), common (25 to 75%), and abundant (>75%). However, the analysis shows that surface textural features present on the grains investigated do not relate to unique environmental processes or conditions. It is very significant to understand the procedures used to identify and classify surface features in different environments (Tickell, 1965). Analyses of the internal surface textural features of grains, combined with mineralogical characteristics such as precipitates, coatings, adhering particles, and surface contaminants, will be very significant in analyses of the micro-environmental process (Pye, 2007). In the present study, 104 grains were analysed from 25 landslides sediment using SEM to understand the process of slope instability and its relationship with mass movement, types of failure, and lithology.
4. Results
4.1 Grain-size analysis
The grain size distribution analysis of the landslide sediments mainly, rock slide, debris slide, and debris fall occurred in the quartzite rocks have a high proportion of gravel, coarse sand, and less percentage of silt. The % of clay concentration was almost insignificant in these sediments. The grain size distribution composition is significant to analyses of slope material which usually determines infiltration capacity, soil moisture condition, water pressure, and cohesive strength. The composition and texture of the sediment show a direct relationship between the proportion of gravel, sand, and silt with the mechanism of slope failures. It is observed that a higher percentage of gravels and coarse sand are present mainly in debris fall and slump types of the landslides, which are rotational slides (Fig. 3). However, rockfall and debris fall a vertical collapse occurred in the quartzite and slate have also a high proportion of gravels.
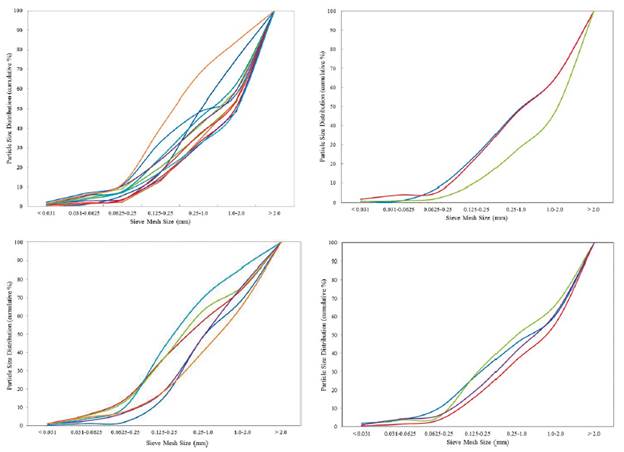
Figure 3 Cumulative Particle size % of the slope material collected from landslides: (a) sediment of debris fall, it has lesser fine particles and high % coarse material, (b) sediment of debris flow, it has lesser % coarse material moderate concentration of fine particles, (c) rockfall sediment has high proportions of coarse particles; and (d) slump sediment composition is similar to debris fall, moderate to high % of coarse particles.
The grain size composition of the debris fall shows a more or less similar pattern to the rockfall with a moderately high concentration of gravel. The investigated sediment samples, except DFW120, have a higher concentration of sand and silt. On further analyses with lithology, conglomerate rock shows a similar grain size composition. The compositions of landslide sediments of quartzite lithology have a similar composition to debris falls. The grain size composition of all the gravity-driven failures, such as debris fall, slump, and rockfall, have similarities in the slope material. These have almost moderate to high concentrations of gravel and a lesser amount of fine particles. The sediment composition of debris fall consists of medium to large gravels and coarse-grained sand. These sediments have the least amount of silt, i.e., less than 9% of the material. However, it has also been observed that a few debris fall sites have a more significant concentration of sand grains and a lesser proportion of gravel and silt.
On examination of the rockfall sediments, having a large part of gravel, it was found that in-situ weathering of slope material dominates the process of failures, having a little run-out distance. A Large proportion of gavels and the least concentration of fine sediments suggest that there is little run-out but are gravity-controlled vertical failures. Similarly, debris falls along the road corridors had a different pattern with lithology. The average concentration of gravel in the sediments of the slump is 41%, debris fall is 40.9%, rockfall is 38.5%, and 25.3% in a debris flow. Thus, indicating a high concentration of fine-grain sediment in the debris flow. Conglomerate rocks have a high proportion of sand and silt concentration in comparison to quartzites. The sediments of debris flow have an average percentage of sand, and silt, but a comparatively higher concentration of clay. The detailed analyses show consistency in grain size composition of slope materials with the type of failures. Except for debris fall and debris flow other failed base rocks, have a similar pattern of particle size composition.
The sediment composition of rockfalls investigated in the area has a predictable pattern with similar grain size composition. Gravel and sand composition of sediments collected from quartzite, slate, and schist-phyllite bedrock have a similar composition as in rockfall and slump. Each type of landslide has a varying composition of grain size composition and texture, hence these results are. Analysis of the failed slope material shows a definite pattern of grain size composition associated with the type of landslide as well as lithology.
Rock falls, debris falls, and slumps occurred in similar lithologies, such as quartzite have similar grain size compositions with few exceptions. Therefore, gravity-driven failures is reveals lithological controls on the sliding mechanism. The analysis concludes that the volume of debris displaced due to landslides has a significant relationship with grain size and lithology in the region.
4.2 Mineralogical composition
The mineral composition and crystallographic structure of slope material in powder form were examined using the x-ray diffraction technique. As given in Supplementary Table 1, quartz mineral is abundant in all the sediment samples collected from landslide sites. However, the concentration of quartz is lesser, mainly, in the conglomerate's rocks. Silicates, carbonates (calcite>>dolomite), and feldspars minerals are also found in a lesser amount in a few samples, whereas traces of gypsum and hematite (Fe2O3) are present in the failed slope materials. It is notable that hematite concentration found in slope material representative of active slide surfaces and discontinuities probably have reactivated landslides.
The higher silica (SiO2) concentrations of minerals are found in quartzite rocks of the LHC rock formations, particularly the Partapnagar brown quartzite which has a 93% SiO2 concentration. The diffraction data reveals that high and thin SiO2 peaks, particularly in quartzite rocks, show a well-crystalline structure and lesser lattice strain. The width of peaks in a particular phase pattern indicates average crystalline size. Large crystalline size gives rise to sharp peaks, while peak width increases as crystalline size decrease. The peak broadening also occurs as a result of variation in' spacing caused by microstrain in the rock. A broad base of peaks shows significant' spacing of molecules. It is often called void space between facies. Peak-to-peak comparison of mineral reflection provides details of the structural deformation process and lattice strain in the diffraction data. Particularly, broader peaks exist in the dolomite rock while quartzite rocks have a narrow and high-rise peak, possibly showing the vast extent of lattice strain. The difference in these peaks appears due to a single phase because the relative intensities vary considerably among different slope materials.
The rock mass strength measured of the landslide sites compared with mineral composition shows that highly fractured and sheared bedrock has weak strength and vice-versa. Figure 4b illustrates the regression value (R2) is 0.34, which indicates a reasonable relationship with mineral composition. However, the SiO2 composition of slope materials and rock strength has a strong correlation; that varies in different lithologies. Quartzite rocks, in particular, have high strength in comparison to dolomite, slate, and schist-phyllite. Comparatively, high rock strength is found in the rockfall and debris fall slope materials. The bedrocks have large cracks, and fractures show weak strength. The slope materials are having alternate bands of quartzite and dolomite strata, are highly weathered, and have poor (14.3MPa) rock strength. It shows a direct relationship between types of lithology and weathering of material related to mineral influences slope failures. The volume of displaced material varies according to the strength of the rock and slope inclination. Slope lithology in failed sites has relatively low rock mass strength, which represents debris flow and debris fall, while sites with high rock mass strength are prone to rockfalls and rock slides.
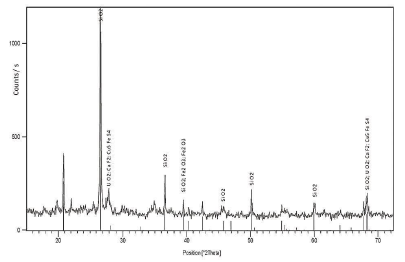
Figure 4 X-ray diffraction of the quartzite rock specimen, showing a sharp and high peak of SiO2 show well defined crystalline structure and lattice strain from the Lesser Himalayan crystalline rocks, using Cu Ka radiation anode
The relative proportions of quartz minerals were subsequently assessed using lithology and SEM analysis. The peak of each sediment sample shows higher variations in the concentration of minerals and phases (Fig. 4). Those rocks are having a more significant proportion of quartz minerals show thin and high peaks in these sediments. Some sediment sample has more than 2000 counts of phases in the samples. Rockfall sediment shows varying proportions of quartz minerals as high as 91 to 94%. Lithologies, with a high concentration of quartz minerals, have greater rock strength in comparison to a lesser concentration of SiO2. Figure 5a illustrates the sediment analysis of schist-phyllite rocks of debris fall (DFL132) and the composition of minerals. It has 74.3% quartz (SiO2), magnetite (Fe2O3) 14.4%, and Dilanthanum Tris (molybdate) 10.4% minerals; Figure 5b presents the composition of debris flow (DFW120) in quartzite lithology.
4.3 SEM Analysis
Meticulous examination of quartz grain micro-surface features done using SEM showed that quartz grains are ambiguous and also challenging to distinguish between morphological processes. The majority of the grains' outlines are angular and sub-angular and may be of glacial and glacial-fluvial origin. These slope material samples were collected from altitudes between 1600-2700m above sea level. Grain outline shows the roundness or angularity of the features of the grain subdivided into angular grains with sharp edges subangular with slightly blunt edges, and rounded grains with smooth edges (Fig. 6). Grain outline mainly relates to the mode of transportation process, distance, time, and up to a certain extent with particle size. Though, it is equally a function of the original grain shape and size from the source rock (Goudie and Watson, 1981; Kleesment, 2009; Costa et al., 2013; Vos et al., 2014). Sub-angular to rounded grains are produced in upper flow regimes as it requires severe abrasion to round the particle edges (Mahaney, 2002). It indicates that flow regimes at this altitude were many times higher in the Bhilangana River as compared to the present. The angular grains' outline occurs in glacial-dominated landscapes where they are crushed, abraded, and plucked in high-energetic subaqueous environments. These areas have limited transport distances of sediment, causing grain breakage without rounding edges (Helland and Holmes, 1997). It indicates that glaciers extended downstream to much lower altitudes in the past to have left these signatures on the quartz grains. The glacial process is mainly dominated by grinding and abrasion during ice transport and glacio-fluvial activity. The digenetic/ alteration in the environment is very widely defined, comprising all processes, mostly chemical, altering the grain surface after deposition and before metamorphism of the source rock (Vos et al., 2014).
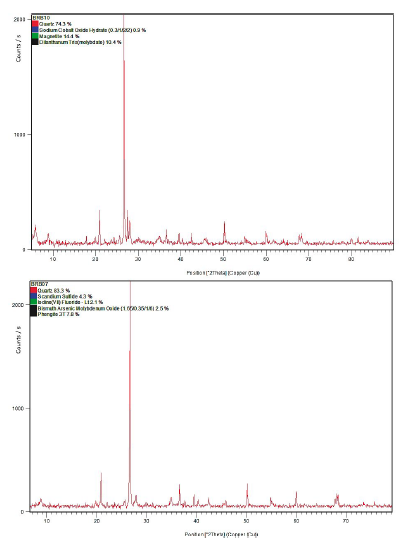
Figure 5 X-ray diffractograms of clay minerals: (A) diffraction peaks of dolomite rocks showing order reflection and broad base, with less SiO2 mineral composition, and (B) well-defined peaks of red quartzite rock, a high concentration of quartz with a mixture of trace minerals.
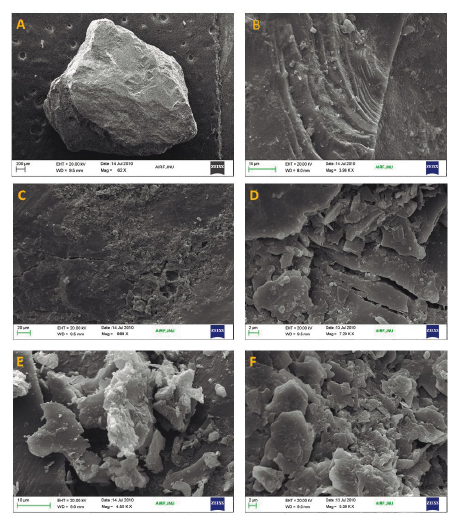
Figure 6 Micrographs showing the surface texture of quartzite rock specimen collected from debris fall, Lesser Himalaya: (A) Grain with conchoidal shape and fractures partly smoothened by abrasion. Probably it is a grain of glacial origin, deposited into glacio-fluvial environment, (B) abundance of arcuate steps and quartz crystal overgrowth, (C) solutions pits calcite overgrowth, (D) fracture plane and mica adhering particles, (E) highly fractured angular facieses of glacio-fluvial sediments with carbonate evaporates, and (F) grooves and conchoidal flacks of schist-phyllite angular flakes and medium cracks.
Morphological features, which occupy a large proportion of grain surface, include conchoidal fractures, multi-stepped striations, grooves, dish-shaped depressions, large pits, ridges, and crystalline overgrowths with or without planar crystalline facies (Supplementary Table 2). Small-scale features that have been identified and measured are small pits and various types of grooves, and mineral precipitates. Usually, the grain surface is characterized by the presence of a vast number of closely spaced pits; and dominant small projections are often defined as consuming a frosted advent, whereas surfaces are having a smaller number of irregularities often denoted to pitted structures. Grain outline is possibly altered by chemical interactions, such as solution and precipitates of minerals. Conchoidal fractures are typically curved and shelllike breakage patterns, of the grains lacking clear cleavage directions. These micro-surface features are the result of powerful impact or pressure on the grain surfaces. Crystal lattice generates a ribbed appearance, cracks, and fractures due to the dominating impact of pressure waves into the grains. Small-scale pits (<1 µm) have often been observed on these fracture planes as mineral insertions, which are weakening the crystal lattice. Hence fractures and cracks developed on the grains (Vos et al., 2014).
Conchoidal fracture planes are occupied by the arcuate and straight steps (Cardona et al., 1997). The depths of these steps are usually varied to several micrometres, with an irregular spacing of successive steps up to 5um. The steps were formed due to the imminence pressures of impacts when the conchoidal fracture plane intersects with the cleavage planes of the quartz crystal. However, these features are inherently associated with conchoidal fracture planes. Meandering ridges are also noticed on the grain surface as the cleavage planes intersect on slightly curved conchoidal fractures.
However, the sediment collected from the landslides is highly crushed, deformed, and metamorphosed in the phase of the orogenetic process of the Himalayas. The small surface features are varying with lithological formations. The grains collected from quartzite rocks are highly weathered due to the mechanical weathering process. The micrograph shows the presence of fractures, crystal overgrowths, adhering particles, arcuate steps, and quartz grains collected from rockfall (Fig. 6d) in the Pratapnagar brown quartzite. The presence of large cracks and fractures, crystal overgrowth, and minor stratification with conchoidal shape and grooves marked on the grain surfaces. Therefore, it indicates that slope failures have occurred due to weak rock mass strength, highly disintegrated minerals, cracks, and fractures (Fig. 6).
Micrographs of quartz grains studied from slump sites in quartzite rock of the Ghansali formations show angular facies, crystal overgrowth, medium size groves with minor fractures, disintegrated granules and foliated structures (Fig. 7). Dolomite rock sites where debris fall has occurred show less disintegrated and angular flakes, but the presence of pits and medium-sized grooves on the surface, indicating active chemical weathering (Fig. 8). Sediments of dolomite and limestone rocks have small to medium pits, grooves, and carbonate precipitate in grain texture. Angular, sub-angular facies, disintegrated flakes, striation, and crystal overgrowth suggest glacio-fluvial transportation of sediment. Micrographs of sediment collected from slate and schist-phyllite rocks show disintegrated angular flakes, fractured rocks, irregular grain shape, and texture. Debris fall and rockfall types of failure occur due to weak lithology and highly weathered surfaces in such rock groups (Fig. 9). The presence of large cracks, medium relief, and crystal overgrowth might suggest sedimentation strain due to seismicity in the region as well. These grains have rounded to a sub-rounded shape, which shows the transportation of unconsolidated materials and deposition below the elevation of 1400 meters in the Lesser Himalayan meta-sedimentaries rock formations. The results of the analysis have compared frequency histograms (Fig. 10).
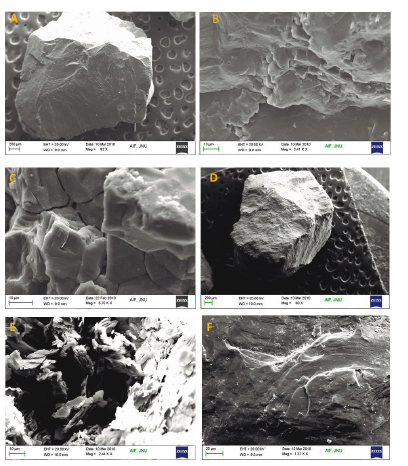
Figure 7 Micrograph of debris flow: (A) sub-angular shape of quartz grain with the abundance of arcuate steps and smooth surface due to abrasion, (B) small irregular pits, medium conchoidal fractures, (C) fine edge fractured amphibole textures with minor cracks, (D) angular outline of grain specimen of rockfall, it has fine edge fractured amphibole textures with minor cracks; fine edge fractured amphibole textures, (E) large conchoidal fractures with minor cracks schist-phyllite platy structures, crystal overgrowth, minor fractures and rounded grooves, and (F) fractured and crystal overgrowth, small pits of carbonate evaporates shows strong chemical weathering environment. It has straight steps with high relief.
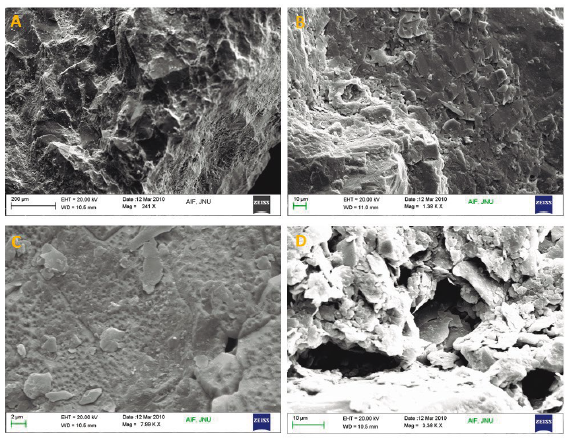
Figure 8 Micrograph of slump sediment: (A) meandering ridges with curved scratches, high relief, (B) fractures plates and siliceous precipitates angular foliated structures of glacio-fluvial origin, (C) presence of large size grooves, sharp edges and highly weathered crystalline structures, disintegrated material of quartzite rocks, and (D) large size cracks and grooves in the amphiboles and small crystal overgrowths. The minerals show characteristics of the physical weathering process with disintegrated minerals of mica-schist, minor fractures, and small crystal growth are persistent.
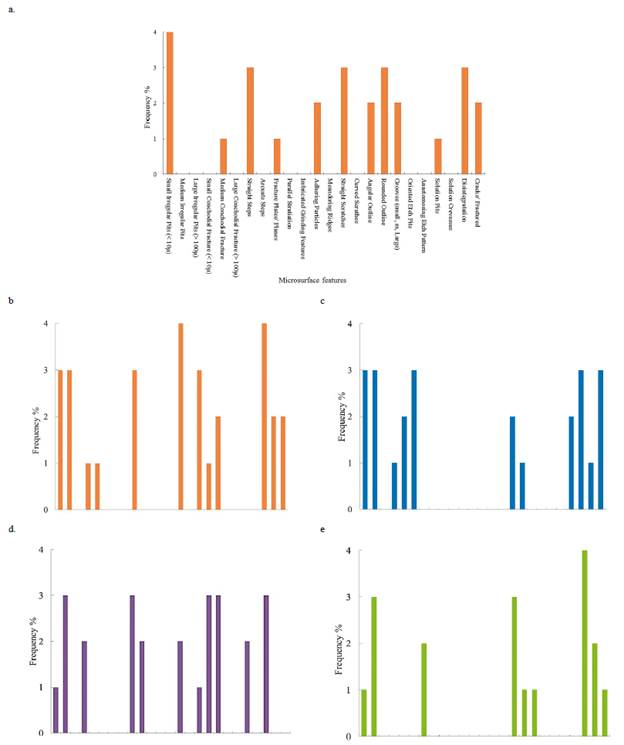
Figure 9 Frequency distribution of quartz grain micro-surface texture presence on the sediment of landslide in the basin: (a) micro-surface features of debris fall sediment DFL298 of conglomerate rock, having both rounded and angular facies, cracks and conchoidal fracture and small solutions pits, presence of adhering particles; (b) debris fall occurred in quartzite rock DFL304, showing conchoidal fractures and solution crevasses, disintegrated surface; (c) debris flow in quartzite rock DFW120, moderately high cracks and fractures, small grooves and irregular pits, rounded outline; (d) rock fall in mixed slate/phyllite rocks RFL308, medium irregular pits, small conchoidal fractures, angular outline, meandering ridges and adhering particles; and (e) slump in quartzite/conglomerate lithology, occurred due to seepage in cracks and joints, showing medium irregular pits, arcuate steps, solution crevasses, cracks and joints (SLM153).
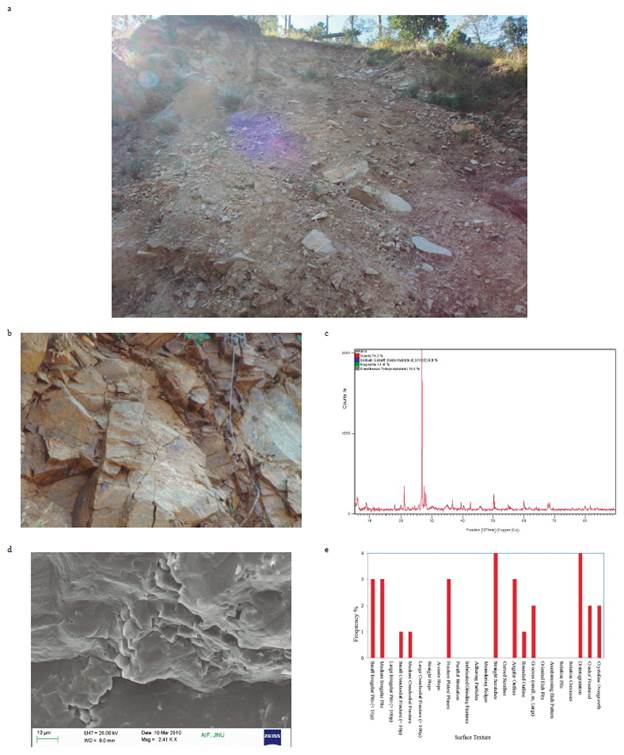
Figure 10 Debris fall (DFL132) occurred in Bhilangana basin: (a) photographed of debris fall showing active slip zone and slide head in the disposed of materials, (b) highly fractured brown quartzite rock of Pratapnagar formation, exposed on the slide head where the slip zone initiated, (c) diffraction peak of clay mineralogy of the sediment collected from the site, showing a sharp and thin peak of SiO2. The mineral composition of the slope material is 74.3% quartz, magnetite 14.4%, Dilanthanum Tris (molybdate) 10.4% and Sodium cobalt oxide hydrate 0.9%. Due to the presence of Fe2O3, chemical dissolution in slope material by rainfall infiltration induced active slip zone at slide head, (d) micrographs showing the fractured presence on the quartz grains as seen in the (b), small pits, adhering particles and sharp flacks, and (e) frequency histograms showing the micro-surface features analyzed from the sediment.
5. Discussion and Conclusion
The grain size distribution, mineral composition, and micro-surface texture analysis indicate a strong relationship with slope failure mechanisms in diverse lithologies. However, the search for a link between slope instability and compositional parameters, such as textural, mineralogical, and micro-surface features, is still a developing field of research (Summa et al., 2013). In the present study, compositional variables and the heterogenetic nature of the data makes the analyses rather complicated.
The grain size distribution of landslide sites shows a definite pattern of material composition and its relationship with the types of landslides. Sediments are having a large proportion of granules, coarse sand, and lesser silt mainly found in rockfall, debris fall, and slump. The medium to fine size silt and sand, with a sufficient proportion of clay composition, influences debris flow, mudflow, and soil creep and are associated with slumps and debris fall in the area. The mineralogical composition of fine sediment fraction analyses shows that variations in the concentration are a cause of landslides (Fig. 11). The high SiO2 composition is significantly related to rock mass strength (Mpa). The sediment sample, which has > 80% of quartz minerals, has rock strength above 30 Mpa (R2 0.43). Further, the rock strength was correlated with the size and volume of landslides and found it was necessary for slope failures that occurred in the lower Himalayan meta-sedimentary rock formations. The fine sediment composition of slope materials and the chemical, mineralogical, and textural compositions of sediment, as well as the study of the process interaction of material involved, are very crucial in influencing the physical-mechanical characteristics of the landslide.
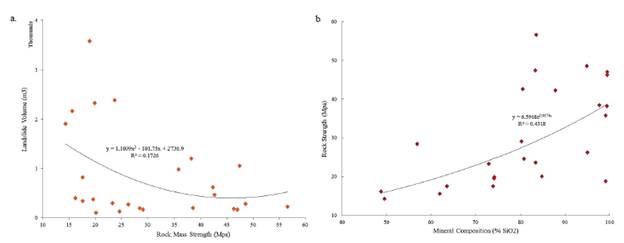
Figure 11 Analysis of landslide pattern and mineral composition (a) relationship between the composition of SiO2 and rock mass strength, and (b) rock mass strength has significantly influenced area and volume of failures.
The Fe2O3 and calcite develop a suitable orientation of particles, thus providing some degree of lubrication, whereas mixed layers are prone to the aggregation of particles in the sub-humid environment where the materials are deposited. Diffraction data of clay mineralogy provided clear foresight of the mineral composition of sediments. The analysis shows that minerals having large' spacing between molecule arrangements, are observed from the weak rock strength and reach the critical limit of tensile strength, causing failures. The results of mineralogical analysis and rock mass strength have a strong relationship and to a certain extent, are supportive in understanding slope failure processes. The quartzite rocks exposed to the weathering process are highly disintegrated, thus reducing rock strength, and causing rockfalls and slumps in the area. The textural changes induced by water chemistry depend on mineralogical composition to a large extent.
The analysis of quartz grain surface textures shows that precipitates of silica and carbonates indicate the influence of regional climate. These areas have undergone various phases of climatic change and tectonic activity in recent geologic history. Hence, the glacial and deglacial processes have played a significant role in the supply of sediments deposited in the river valleys. The debris flows sediment samples collected near Ghuttu village show angular pattern, stairation surface, and grooves indicative of a glacial environment. The analyses conclude that slope material is deposited during a glacial advance. During the monsoon season, debris flow, debris fall, and debris avalanches frequently occur in these unconsolidated lithofacies due to lubrication. Grain textures in these areas have undergone a lot of geodynamic processes during recent geological history through neo-tectonics.
SEM analysis has added many advantages to geomorphology, including the determination and origin of depositional landforms, the source of sediments, the energy of the environment and processes of diagenesis, weathering, and development through time. It provides details of the origin of fine silt and clay particles in the geological column, fracture-abrasion mechanisms in the field and laboratory, and analysis of grain modifications under different weathering regimes. The microscopic study of quartz grain is used to understand texture, pattern, internal morphology, and weathering pattern prevalent in the area, and its correlations with slope instability. It provides a close relationship with rock strength and the volume of displaced material. The study of microtextures is, therefore, a useful technique to reconstruct the sedimentary history of the displaced materials, and should be judiciously used more often for studies of clastic sediments.
The present study is an experiment that analyses the significance of sediment characteristics in the slope instability processes. It also provides new stimuli to further site-specific investigations such as grain size distribution, mineralogical and micro-surface texture, and their relationships. The mineral composition is closely related to producing sliding surfaces and discontinuities in the slope materials. The present study finds that the slope instability process is controlled by site-specific material composition, the presence of minerals and the depositional environment. The geotechnical parameters such as liquid limit, plastic limit, and angle of shearing resistance on the sliding surface may require such analysis to determine the relationship with the failure mechanism. However, it is distinguishing between the cause and effect even though some risk factors were identified, which are inducing slope failures. The present study will be very useful for the site-specific slope instability analysis and selection of appropriate stabilization measures. The in-depth analyses of these parameters will contribute to the knowledge of the geotechnical characteristics of landslide investigations.