1. Introduction
Navigating and surviving in a constantly changing world of complex stimuli requires fast and precise processing of sensory information. This process, which is strongly modulated by experience, requires the integration of the bottom-up intake of sensory information and topdown regulation by which context information modifies the perception of stimuli (Broadbent, 1958; Hanson et al., 2021; Hernández-Peón et al., 1956; Rauss & Pourtois, 2013). Top-down regulation is achieved in part by experience-dependent neuromodulation of the cortical and subcortical regions by several neuronal afferents that modify neuronal excitability from milliseconds to hours (Bouret & Sara, 2004; Doucette & Restrepo, 2008; Lee & Dan, 2012; Nunez-Parra et al., 2020; Rajkowski et al., 2004; Yang et al., 2017). Moreover, it has been proposed that sensory discrimination leading to adequate decision-making requires the simultaneous modulation of several brain regions processing sensory, motor, and cognitive information (Grossberg et al., 2016).
A neuromodulatory center that has been proposed as a candidate for this role is the basal forebrain (Hasselmo & Sarter, 2011; Khalighinejad et al., 2020; Lin & Nicolelis, 2008; Richardson & DeLong, 1990; Voytko et al., 1994; Wilson & Rolls, 1990). The basal forebrain (BF), located ventrally in the anterior portion of the mammalian brain, projects to several brain regions that have been involved in a wide array of cognitive functions such as attention, motivation, arousal, reinforcement responses, and memory (Agostinelli et al., 2019; Buzsaki et al., 1988; Chaves-Coira et al., 2018; Gritti et al., 1997; Han et al., 2014; Hangya et al., 2015; Shi et al., 2015). Interestingly, it is also known to play an important role in modifying sensory perception and particularly olfactory perception, where its massive cholinergic projections to olfactory centers control olfactory coding from sensory discrimination to decision-making and attention control (D’Souza & Vijayaraghavan, 2014). The multiple cognitive and sensory functions the BF regulates, strongly suggest that it may be acting as a coordination center for sensory processing and adequate decision-making.
However, the mechanisms behind BF modulation are not well understood. In this work, we will review the latest studies that have shed light on the role of BF modulation on rodent olfactory circuits. We will focus on research that has been performed in vivo and in awake, behaving animals to further link the role of the BF in olfactory coding and decision-making.
Importantly, alterations in sensory sensitivity and filtering are prevalent symptoms in patients with neurological disorders such as autism spectrum disorder (ASD) (Ashwin et al., 2014; Bennetto et al., 2007; Boudjarane et al., 2017; Cascio et al., 2015; Chung & Son, 2020; Dudova et al., 2011; Shah & Frith, 1983; Stevenson et al., 2019; Suzuki et al., 2003; Yu & Wang, 2021). It is unclear, though, if the differences in sensory processing are influenced by anomalies in the mechanisms of top-down neuromodulation, such as neuromodulation of BF, despite the evidence showing anatomical and functional differences of this brain region (Bauman & Kemper, 1985; Kemper & Bauman, 1998; Riva et al., 2011; Wegiel et al., 2014). Hence, we will summarize in this revision anatomical and functional abnormalities found in the BF and cholinergic system of individuals with ASD and conclude by proposing that this relationship could open new windows of opportunity to explore the neurobiology underpinning of ASD sensory dysfunction.
2. The Structure and Function of the Basal Forebrain
The BF is a region in the vertebrate brain that includes several nuclei, such as the medial septum (MS), ventral pallidum, vertical and horizontal limbs of the diagonal band of Broca (VDB and HDB), magnocellular preoptic nucleus (MPCO) substantia innominata, and peripalladal regions (Gritti et al., 2006; Záborszky et al., 2018). These nuclei are groups of neurons that are heterogeneous in their morphology, projections, and neurotransmitter content, including cholinergic, GABAergic, glutamatergic, and peptidergic neurons. Despite accounting only for about 5% of the total neuronal population of this brain region, cholinergic neurons in the basal forebrain are a major source of acetylcholine (Ach) in the brain (Gritti et al., 2006; Záborszky et al., 2018). There are extensive neuronal projections to and from the basal forebrain. Neurons in the basal forebrain receive input from the dorsal and ventral striatum, hypothalamus, amygdala, brain stem tegmentum, and the cortex with different innervation densities (Do et al., 2016; Zaborszky & Gombkoto, 2018). Outputs from the BF are myriad, including the hippocampus, basolateral amygdala, hypothalamus, and much of the neocortex (Alitto & Dan, 2012; Semba, 2000).
Due to these wide-reaching projections, the basal forebrain has traditionally been attributed to a uniform and diffuse neuromodulatory role (D’Souza & Vijayaraghavan, 2014; Saper, 1987). However, anatomical and functional descriptions have revealed highly organized topographic patterns in its projections, suggesting that different areas of the BF may discreetly modulate distinct neuronal processes (Chaves-Coira et al., 2018; Gielow & Zaborszky, 2017; Nunez-Parra et al., 2020; Zaborszky, 2002; Zaborszky et al., 2015).
The BF diffuse connectivity makes this structure an interesting candidate for a main top-down modulator playing a principal role in decision-making. This function would likely complement the role of other structures involved in this process such as the prefrontal cortex, amygdala, and hippocampus, among others (Gupta et al., 2011; Khalighinejad et al., 2020; Yu & Frank, 2015). Indeed, acetylcholine from the BF has been implicated in several key cognitive processes, such as learning and memory, attention, wakefulness, responses toward reward and punishment, reward timing, and motivation (Devore & Linster, 2012; Goard & Dan, 2009; Hangya et al., 2015; Hasselmo & Sarter, 2011; Klinkenberg et al., 2011; Laszlovszky et al., 2020; Luchicchi et al., 2014; Mark et al., 2011; Minces et al., 2017; Steriade, 2004). Notably, the BF also plays a fundamental role in sensory processing and discrimination across sensory modalities, including vision (Alitto & Dan, 2012; Goard & Dan, 2009; Minces et al., 2017), audition (Leach et al., 2013), touch (ChavesCoira et al., 2018; Chaves-Coira et al., 2018) and olfaction (Nunez-Parra et al., 2013) by sharpening neuronal response to sensory stimuli regulating excitatory and inhibitory neurons, decorrelating neuronal activity, improving the signal/noise ratio and sensory encoding (Guo et al., 2019; Pinto et al., 2013; Thomson et al., 2014).
Among the sensory systems, olfaction offers unique features, which make it a very appealing experimental model system to study the role of BF modulation on sensory processing. In particular, there are heavy projections from the BF to olfactory circuits such as the olfactory bulb, and the piriform and entorhinal cortices, mostly stemming from the HDB and the MCPO (Hur & Zaborszky, 2005; Zaborszky et al., 2012). Although BF cholinergic projections have been the most studied, GABAergic and glutamatergic neurons also play an increasingly appreciated role (Böhm et al., 2020; Hanson et al., 2020; Lin et al., 2015; Nunez-Parra et al., 2013). From an experimental point of view, the olfactory system exhibits neuronal circuits that are highly organized, with clearly identified layers of inputs and output of sensory information (Burton, 2017; Gheusi et al., 2013) that are easily accessible to experimenters (Brunert & Rothermel, 2019). Additionally, compared to others, the olfactory system exhibits an anatomically "simpler" neuronal circuitry, which makes it an ideal model to pair electrophysiological studies and the role of neuromodulation of neuronal activity with behavior (Doty, 1986). Subsequently, as we will review in this article, several groups have used the olfactory system to study the role of cholinergic and GABAergic neurons from the BF modulating olfactory processing.
3. Olfactory Coding
Olfaction is one of the most ancestral senses and a sophisticated neural circuit extracts accurate relevant information from the environment to elicit behaviors such as space orientation, mating, aggression, parenting, predator identification, and food location (Hoover, 2010). Olfactory transduction starts in the olfactory sensory neurons located in the olfactory epithelium of the nose, specifically in the cilia, where olfactory receptors are expressed (Figure 1A; Bronshteín & Minor, 1977). Interestingly, each olfactory sensory neuron expresses one specific olfactory receptor out of around a thousand distinct receptor genes found in mice -humans express more than 400 (Olender et al., 2012)-. Olfactory receptors can be activated by different odorants (at different sensitivities) and a particular odorant can activate more than one receptor (Buck, 1992; Malnic et al., 1999). This arrangement generates a combinatorial code where subsets of olfactory neurons are activated by the olfactory stimulus. This biological solution is very efficient as it allows for thousands or even millions of odors to be perceived only with a limited number of olfactory receptors. Downstream, olfactory sensory neurons expressing the same receptor, project to one particular spot in the olfactory bulb (OB), known as a glomerulus. The glomerulus is a functional unit that includes a diverse array of juxtaglomerular cells like the inhibitory periglomerular cells (PGs), the axon terminal of sensory neurons, and the dendritic branch of the principal neurons, known as the mitral and tufted cells (Gire & Schoppa, 2009). Since most smells are found in nature as part of a mixture, olfactory sensory neurons subpopulations activate several glomeruli, creating an activation pattern in the olfactory bulb for each odor (Friedrich & Korsching, 1997; Johnson & Leon, 2007; Ressler et al., 1994; Soucy et al., 2009; Spors & Grinvald, 2002; Uchida et al., 2000; Vassar et al., 1994) that can change depending on context (Kudryavitskaya et al., 2021). Mitral (MCs) and tufted cells extend a single dendrite to a unique glomerulus (Malun & Brunjes, 1996) and immediately project to the olfactory cortex (Figure 1C; Igarashi et al., 2012; Nagayama et al., 2010; Price, 1973), bypassing thalamus. In other words, the sensory input is only two synapses away from the cortex. The olfactory cortex is further composed of several structures such as the piriform cortex, anterior olfactory nucleus, olfactory tubercle, entorhinal cortex, and the lateral portion of the amygdala (Price, 1973).
In the bulb, the firing of MCs is regulated mainly by inhibitory PG neurons and granule cells (GCs) (Burton, 2017; Gire & Schoppa, 2009; Schoppa et al., 1998). PGs, located in the glomerular layer, are heterogeneous and can be activated directly by the glutamate released by the olfactory sensory neurons or indirectly by sensoryevoked excitatory input of other juxtaglomerular neurons (Shao et al., 2009). They are broadly tuned and would cooperate in the refinement of the activity patterns in the olfactory bulb (Arruda et al., 2013; Tan et al., 2010). On the other hand, the axonless GCs located in the granule cell layer are the most numerous neuronal type in the olfactory bulb and most research has been focused on the GC-MC interaction. The dendrites of GCs synapse onto the lateral dendrites of MC through a reciprocal dendrodendritic synapse, where the MC release glutamate onto GCs, and they, in turn, release GABA back onto the MC (Isaacson & Strowbridge, 1998; Jahr & Nicoll, 1982; Rall et al., 1966; Schoppa et al., 1998). Other type of inhibitory GABAergic interneurons in the OB are deep short-axon cells (dSAC). These extend wide ramifications across the bulb layers, playing a relevant part as they regulate the OB excitability (Burton et al., 2017; Eyre et al., 2008; Sanz Diez et al., 2019). The olfactory bulb is believed to transform sensory information, performing a series of neuronal computations before transmitting it to the cortex, such as normalization, contrast enhancement, and sensitivity regulation (Devore & Linster, 2012). Interestingly, the perceptual olfactory response does not only depend on which MCs are activated, but also by their activation latencies. The first ones appear to carry information about the odor identity (Chong et al., 2020; Wilson et al., 2017).
Due to the combinatorial nature of the olfactory network information processing, experimental evidence and computational models suggest that there are two properties that can also contribute to olfactory coding: sparseness and synchronization (de Almeida et al., 2013; Doucette et al., 2011; Gill et al., 2020). A response is sparse when a single neuron is active, while it has minimal sparseness when all cells have the same activity in response to a stimulus. Thus, if a reduced number of MC respond to a determined olfactory stimulus, the amount of odors that can be coded by the network increases (as opposed to, for example, when the majority of MCs respond to a stimulus leading to a very precarious number of combinatorial operations). The increase in sparseness in odor representation in the OB will then be optimal for information decoding by the pyramidal cortical neurons. Moreover, MCs diffusely project to neurons in the piriform cortex with no apparent topography (Ghosh et al., 2011). A particular MC projects to multiple pyramidal neurons and one pyramidal neuron receives inputs from several MCs (Wilson & Sullivan, 2011) with random connectivity, although recent data has shown that there might be a structured relationship between bulbar outputs and cortical connectivity (Chen et al., 2022). Along the same line, it had been widely thought that odors activate spatially distributed ensembles with an apparent lack of topographical organization, although recent evidence is suggesting that the cortex might cluster together representation for odors that share chemical attributes (Pashkovski et al., 2020). In any case, studies have shown that a given pyramidal neuron can only be activated by the combined activity of multiple glomeruli, not by single individual pathways (Apicella et al., 2010), corroborating the idea that asynchronous inputs may not be able to excite pyramidal neurons and that synchronization of MC output might be required.
Importantly, the temporal dynamics of information processing are particularly relevant in olfaction. Oscillations at different frequencies are thought to carry information associated with olfactory processing, specifically learning and discrimination (Kay, 2005; Nusser et al., 2001). For instance, odorants come into the nasal cavity through inhaled air and consequently, changes in MC firing rate are coupled to sniffing in what is known as the theta frequency (2-12 Hz) (Cang & Isaacson, 2003). This oscillatory activity is relevant in the decision-making process and it has been shown that odor identity (‘what is the odor?’) is temporally coded in the redistribution of firing activity within the respiratory cycle -and not a change in firing rate- (Gire et al., 2013; Gschwend et al., 2012; Uchida et al., 2014). Neuronal oscillations in the gamma range (40-90 Hz) are also found in the OB and are thought to originate in the GC-MC interaction during which neurons synchronize (de Almeida et al., 2013; Fukunaga et al., 2014; Lagier et al., 2004; Nunez-Parra et al., 2014; Nusser et al., 2001). Importantly, the coherence between spike timing in MCs and this gamma frequency band are also suggested to carry information about odor identity in the OB (Lin et al., 2015). Therefore, olfactory information is coded by complex temporal patterns of action potential firing strongly modulated by respiration and network oscillatory activity. Indeed, regulating the spatiotemporal scope of MC activity is crucial for olfactory discrimination (Fukunaga et al., 2014; Gheusi et al., 2013; Xiong & Chen, 2002; Yokoi et al., 1995), a tight regulation that is mainly achieved through top-down control of bulbar and cortical neurons.
Accordingly, the OB network is strongly regulated by centrifugal afferents (Boyd et al., 2012; Matsutani & Yamamoto, 2008; Oswald & Urban, 2012), such as cholinergic and GABAergic afferents from the BF -as can be seen in Figure 1B- (Luskin & Price, 1982; Salcedo et al., 2011; Senut et al., 1989; Shipley & Adamek, 1984; Záborszky et al., 1986; Záborszky et al., 1986). In vitro studies and computational modeling have suggested that Ach plays a role in regulating neuronal excitability, synchronization and network sparseness, and oscillatory power (D’Souza & Vijayaraghavan, 2014; de Almeida et al., 2013; Devore & Linster, 2012; Li & Cleland, 2013; Villar et al., 2021). However, despite the crucial role of Ach and the BF in olfactory processing, its physiological role in vivo remains to be described in full. Here we will focus on those studies.
4. The Role of Basal Forebrain Cholinergic Modulation onto the Olfactory Bulb
Earlier works into the role of BF projections in the OB circuitry in anesthetized animals and brain slices, along with pharmacological approaches, showed some conflicting results.
Most of the BF projections to olfactory circuits arise from the HDB and the MCPO (Hur & Zaborszky, 2005; Zaborszky et al., 2012), but other cholinergic projections to the olfactory bulb arise from the substantia innominate (SI) and medial septum and VDB, as well as GABAergic projections from the ventral palladium (Do et al., 2016; Li et al., 2018; Moyano & Molina, 1982; Záborszky et al., 1986). However, their bulbar targets remain unclear (Figure 1B). In general, the distribution of these inputs appears to be layer-specific, with cholinergic and GABAergic afferents neurons mostly innervating the granular and glomerular layers (Figure 1C; D’Souza & Vijayaraghavan, 2014; Gracia-Llanes et al., 2010; Li et al., 2018; Nunez-Parra et al., 2020). Similarly, the BF receives abundant afferents from the OB and olfactory cortices, with the strongest innervation reaching the HDB/MCPO/SI complex (Zheng et al., 2018).
Ach transduction is mediated through two distinct receptor types: nicotinic and muscarinic receptors. The nicotinic receptors are ligand-gated ion channels that allow the influx of sodium and calcium ions and the outflux of potassium ions. In neurons, nicotinic receptors can be hetero or homomeric, consisting of the α and β subunits. On the other hand, muscarinic receptors are G-coupled metabotropic receptors that can be subdivided into five different subtypes (M1-M5). Each of these subtypes can be associated with excitatory (M1, M3 y M5) or inhibitory (M2 and M4) intracellular signaling pathways. Specifically in the OB, cholinergic receptors are neuron and layer dependent. Nicotinic receptor (nAchR) of the α3β4*-nAchR and the α4β2*nAchRsubtypes are expressed in the glomerular layer, probably in the apical dendrite of MCs, while muscarinic subtypes (M1 and M2) are found in deeper layers of the bulb in particular where the dendrodendritic synapse between mitral and GC is located (D’Souza & Vijayaraghavan, 2014), but also in PGs (Liu et al., 2015). In addition, dSACs receive projections from the HDB that co-release both GABA and acetylcholine, suggesting a very specific mechanism of neuromodulation in the OB (Case et al., 2017). Interestingly, modulation of the olfactory network by Ach and GABA is a centrifugal action mostly, but not exclusively, produced over inhibitory cells of the olfactory bulb network, that modulate the activity of the MCs (Case et al., 2017; NunezParra et al., 2013; Smith et al., 2015; Villar et al., 2021).
In vitro electrophysiological studies recording OB neuronal responses in slices have suggested that cholinergic agonists directly depolarize MCs and GCs (Smith & Araneda, 2010) or that they depolarize MCs and inhibit GCs (Castillo et al., 1999; Fletcher & Chen, 2010). Due to the overlapping neuronal receptor expression, the cholinergic effect has been found to be bidirectional in some scenarios. For example, in vitro analysis of the effect of muscarinic agonists in the bulb has shown that it inhibits MCs while it generates a dual effect on GC: hyperpolarization and depolarization through M2 and M1, respectively, and discrepancies between the effects of HDB lesions and anticholinergic drug administration in olfactory learning have also been found (De Rosa et al., 2001; De Rosa & Hasselmo, 2000; Robinson et al., 2011; Roman et al., 1993; Smith et al., 2015). Fortunately, thanks to techniques that have emerged in the last decades, such as calcium imaging and optogenetics, much more detailed and precise analyses have helped to uncover the role that the BF plays in olfactory processing.
Indeed, as shown by calcium imaging and electrical stimulation of the HDB in anesthetized animals, one group has found that cholinergic MC response was actually bimodal (Bendahmane et al., 2016). This was measured through wide-field imaging of the light emitted by the fluorescent calcium indicator GCaMP2, exogenously expressed in the MCs in the OB of transgenic mice. Recordings were made in the glomerular layer and the responses were measured from the apical dendrites of MCs. Stimulation of the HDB resulted in modulation of neuronal sensitivity, enhancing responses to low concentrations of odorant, and inhibiting responses to high odorant concentrations. Moreover, using specific agonists and antagonists applied to the bath dilution to the OB surface, they showed that the augmented sensitivity to weaker odor inputs was mediated by the muscarinic receptor M2. On the other hand, the decrease in sensitivity to stronger odor inputs was mediated by nicotinic receptors (Bendahmane et al., 2016). Their findings are in line with work performed in brain slices that reported that Ach can inhibit GCs and PGs through muscarinic receptors leading to MC disinhibition (Liu et al., 2015; Smith et al., 2015), which could underpin the resulting increase in sensitivity to weaker olfactory inputs. On the other hand, the decrease in sensitivity to stronger inputs can be explained by in vitro work that found that glomerular application of Ach caused a rapid and brief nicotinic receptor-mediated excitation of MCs, promoting glutamate release onto the glomerulus. The increase in glutamate can activate PGs that will in turn inhibit MCs, adding an inhibitory bias to the circuit (D’Souza & Vijayaraghavan, 2012, 2014). PG-driven feedback inhibition onto MC could explain the decrease in sensitivity to stronger inputs.
Even though a pharmacological approach such as the one described above gives hints on the role of the cholinergic neurons of the BF regulating the OB circuitry, electrical stimulation unavoidably recruits other BF afferents to the bulb, such as the GABAergic projection neurons. Optogenetic allows for the expression of light-sensitive ion channels in particular neuron types and subsequent precise spatiotemporal stimulation of these neurons through optic fibers (Boyden et al., 2005). This optogenetic approach, along with newer recording instrumentation on awake, behaving animals, has helped to further elucidate some aspects of basal forebrain modulation in the processing of odor information (Table 1). One group used transgenic mice expressing the light-sensitive channelrhodopsin-2 (ChR2) driven by the choline acetyltransferase (ChAT) promoter in their cholinergic neurons (Ma & Luo, 2012). The stimulating optic fiber was placed in the HDB of the basal forebrain, while the recording electrode was placed in the olfactory bulb during olfaction in an anesthetized animal. This setup allowed for the selective stimulation of cholinergic neurons in the HDB while simultaneously recording the activity in the OB as the individual was passively exposed to an odorant. The results showed that cholinergic input into the olfactory bulb differentially modulated neuronal activity, enhancing neuronal activity in some contexts while inhibiting it in others. They found that odorant-evoked activity with simultaneous activation of BF cholinergic neurons sharpened the tuning curves of MCs, meaning that their responses became more specific to a particular odorant, while it suppressed spontaneous activity in GCs, PGs, and MCs. Direct Ach release can activate GCs through muscarinic receptors. That general increase in GC excitability will be complemented during odorant-evoked activity with a second phenomenon called GC-mediated lateral inhibition (Shepherd et al., 2007). Lateral inhibition is the process by which a GC, which is strongly activated by a MC, depolarizes releasing GABA onto MC, projecting to neighboring glomeruli. The stronger the activation of the MC, the higher the possibility of GCs laterally inhibiting neighboring MCs. Consequently, the readout of cholinergic afferents stimulation will be input dependent resulting in the sharpening of MCs receptive fields. In addition, activating cholinergic projecting from out from the HDB includes those innervating regions of the brain other than the OB, such as the entorhinal cortex, hypothalamus, and piriform cortex (Zaborszky et al., 2012), and electrical stimulation of the HDB has been shown to enhance olfactory cortex neuron activity (Linster et al., 1999), which could indirectly modulate bulbar GC-MC interaction. This modulation could occur through pyramidal excitatory feedback projections that terminate onto GCs in the OB, meaning that activation of pyramidal neurons could result in the activation of GCs increasing bulbar inhibition onto MCs (Boyd et al., 2012; Matsutani, 2010). This odor-induced feedback would also sharpen MC responses by inhibiting weak responses. If this is the case, in response to olfactory inputs, MCs activated by weakly activated glomeruli would be inhibited, while MCs belonging to strongly activated glomeruli will be excited, increasing the signalto-noise ratio and enhancing olfactory discrimination.
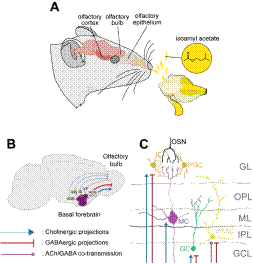
Note. (A) Volatile olfactory signals, such as isoamylacetate, reach the olfactory epithelium in the nasal cavity. Olfactory sensory neurons (OSN) located in the epithelium transduce the information and transmit it to the olfactory bulb in the brain. From there signal is sent downstream to different brain regions like the olfactory cortex. (B) The basal forebrain is located in the ventral brain and is composed of several nuclei that project their axons to most of the brain. Here we depict the ones arising from the HDB which sends cholinergic (blue lines) and GABAergic (red lines) projections to the olfactory bulb. We also depict cholinergic projections from the VDB, MS, SI; as well as GABAergic projections from the VP. The BF also projects to the olfactory cortex (not shown) (C) Diagram of the bulbar neuronal circuit. Axonal terminals of OSN terminate in the glomerulus where they synapse onto the apical dendrites of mitral cells (MC). Inhibitory neurons, the periglomerular (PG), granule cells (GC) and deep short axon cells (dSAC) regulate mitral cells output. In the diagram, the targeted layers of BF GABAergic and cholinergic projections are shown. VP: ventral pallidum; MS: medial septum; VDB: vertical diagonal band of Broca; HDB: horizontal diagonal band of Broca; SI: innominate substance; GL: glomerular layer, OPL: outer plexiform layer; ML: mitral cell layer; IPL: inner plexiform layer; GCL: granule cell layer.
Figure 1 Olfactory System and Neuromodulation of Basal Forebrain onto Olfactory Bulb
Table 1 Compilation of Research Studying the Role of BF in Olfactory Coding In Vivo
Reference | Strains | Main techniques | Drugs used | ChR expression | Light stimulation | Recording site | Results |
Rosin et al., 1999 | Wistar rats | Voltage-sensitive dye RH 795 expressed in the PC OB was electrically stimulated BF was electrically stimulated | - | - | - | PC | ↓ PC activity to electrical stimulation of the OB if it was preceded by electrical stimulation of the BF |
Ma et al., 2012 | Mouse ChAT-ChR2 | Optogenetics | - | BF Cholinergic neurons | BF | OB | ↓ OB spontaneous activity MCs sharper tuning curves |
Nunez-Parra et al., 2013 | Mouse GAD65-Cre | Optogenetics | - | BF GABAergic neurons | OB | OB | ↓ GCs activity (light stimulation over axons of HDB in OB) |
Mouse GAD65-Cre | DREADD (hM4Di) | CNO | - | - | - | ↓ Odor discrimination between similar odorants (hab/dishab task) | |
Rothermel et al., 2014 | Mouse ChAT-Cre, ChAT-ChR2 | Optogenetics | - | BF Cholinergic neurons | OB | OB | ↑ MCs activity ↑ MC activity during inhalation |
Bendahmane et al., 2016 | Mouse GcaMP2 - Kv3.1 prom | Electrical stimulation of HDB during odorant presentation in anesthetized animals | Scopolamine Ecamylamine AF-DX116 Oxotremorin, McN-A 343 | - | - | OB by calcium imaging | ↑ MC activity to low concentrations of odorant through muscarinic receptors ↓ MC activity to high odorant concentration through nicotinic receptors |
Devore et al, 2016 | Long Evans Rats | Recordings | - | - | - | BF | ↑ or ↓ BF activity during spontaneous investigation ↑ or ↓ BF neuronal activity during a 2 AFC behavioral task (during trial initialization and odor presentation) |
B÷hm et al, 2020 | Mouse GAD65-Cre | Optogenetics | - | BF GABAergic neurons | OB | OB | ↓ MC spontaneous activity ↑ MC odor-evoked activity and signal filtering |
Mouse ChAT-Cre | Optogenetics | - | BF Cholinergic neurons | OB | OB | ↑ MC spontaneous activity ↑ MC activity during inhalation ↑ Odor-evoked MC activity | |
Hanson et al., 2020 | Mouse Vgat-Cre Vgatf/f | Optogenetics | - | BF GABAergic neurons | OB | OB | ↑ Survival and integration of abGCs |
Nunez-Parra et al., 2020 | Mouse ChAT-ChR2 | Optogenetics | - | BF Cholinergic neurons | BF | BF | ↑ or ↓ BF neuronal activity during a go-no go behavioral task (during trial initialization, odor presentation and reward delivery) ↑ or ↓ BF cholinergic neurons during a go-no go behavioral task (during trial initialization and odor presentation) |
Hanson et al., 2021 | Mouse | Fiber photometry using choline sensitive indicator GAch2.0 | - | - | - | BF | ↑ Cholinergic tone during a go-no go task (during odor presentation and reward seeking). ↓ after reward delivery |
Mouse GAD 65-Cre | Fiber photometry using calcium indicator GCaMP6M | - | - | - | BF | ↑ BF GABAergic neurons activity during a go-no go behavioral task during odor presentation and reward seeking). ↓ after reward delivery |
Note. ChR: Channelrhodopsin; PC: piriform cortex; OB: olfactory bulb, mAchRs: muscarinic acetylcholine receptors, BF basal forebrain; MCs: mitral cells; GCs: granule cells; abGCs: adult-born granule cells; 2 AFC: two alternative force choice. Up arrow (↑) and down arrow (↓) indicate increase and decrease, respectively.
It remained unclear, though, how cholinergic inputs inhibited the spontaneous activity of all bulbar neurons. However, the experimental setup led to the stimulation of cholinergic neurons in the BF, which exhibit axon collaterals that terminate onto long-range cortically, projecting GABAergic neurons, which in turn express nicotinic and muscarinic receptors (Böhm et al., 2020; Yang et al., 2014; Zaborszky & Duque, 2000). Activation of cholinergic BF neurons could activate cortical GABAergic projections that terminate in the GC and glomerular layer, inhibiting GCs and PGs (Gracia-Llanes et al., 2010; Nunez-Parra et al., 2020). How MCs were inhibited remains to be clarified, but it could also be an effect of indirect BF GABAergic neurons activation as we will discuss later (Böhm et al., 2020).
Yet, it appears that cholinergic projections directly from the HDB into the olfactory bulb add a general excitatory bias, enhancing both spontaneous and odorevoked activity of MCs (Böhm et al., 2020; Rothermel et al., 2014). This was shown in an identical fashion to Ma and Luo’s experiment, the only difference being the placement of the stimulating optic fiber. Here, optogenetic stimulation was directed to the olfactory bulb, to stimulate only the axons of the cholinergic neurons terminating into OB neurons (Böhm et al., 2020; Rothermel et al., 2014). This experiment demonstrated that cholinergic release from BF fibers onto the OB excited MCs regardless of odor presence. Interestingly, they also showed that when the odor-evoked response was coupled to inhalation, and hence to theta respiratory mediated oscillations, MCs activity increase was higher. Attention-dependent cholinergic inputs onto MCs would increase the basal firing rate of these neurons, augmenting it even further in response to odors. Additionally, if a MC is already firing, an odor could not only modify its firing rate but also the timing of spiking activity. In this scenario, synchronization between MC’s firing and theta oscillations will have an impact that would not be possible if MCs were not firing in the first place (D’Souza & Vijayaraghavan, 2014).
Thus, Rothermel and collaborators were able to discriminate between direct effects that increased OB activity and indirect effects (possibly through cortical feedback or activation of GABAergic projection circuits) that lead to bidirectional responses and enhanced odor discrimination after BF cholinergic neurons stimulation (Rothermel et al., 2014). Additionally, we believe that the discrepancies between the work of the Rothermel and Bendahmane groups could arise in part since the latter was studying the superficial layers of the bulb using different stimuli concentrations. Rothermel and collaborators recorded deeper layers of the bulb and used a supra-threshold odorant concentration throughout their experiments, probably engaging MC activation through PG feedback inhibition and GC lateral inhibition. How acetylcholine regulates concentration-dependent MC responses still needs further clarification.
5. The Role of GABAergic Projections from the Basal Forebrain to the Olfactory Bulb
Although BF cholinergic influx into the olfactory bulb has been the most extensively studied, GABAergic projections also play a significant role in olfactory modulation, as shown in recent studies. Firstly, Nunez-Parra and collaborators selectively labeled GABAergic neurons of the BF with ChR2 (2013). Through in vitro
analyses of brain slices, they showed that optogenetic stimulation of GABAergic terminals in the OB extending from the HDB inhibited GC responses, probably resulting in the disinhibition of MCs in the OB (NunezParra et al., 2013). This interaction seems to be important in olfactory processing, as inhibition of GABAergic neurons in the basal forebrain led to impairment in discrimination in a habituation/dishabituation task. In this task, animals are repeatedly presented with an odor and the investigation time is measured. Once the animal has habituated, a novel odor is presented. If the animal perceives the new odor as distinct, investigation time will rise again (dishabituation). Interestingly, inhibition of GCs is required for fine olfactory discrimination, since animals dishabituated to a dissimilar odorant, but could not distinguish between structurally similar ones (Nunez-Parra et al., 2013).
A more recent optogenetic approach used the same strategy described above to selectively express ChR2 in BF GABAergic neurons to study their role during olfaction in anesthetized animals (Böhm et al., 2020). They showed that optogenetic activation of GABAergic terminals into the olfactory bulb (mostly from the HDB) modulates MC activity, suppressing spontaneous firing and increasing odor-evoked responses, as measured by extracellular recordings in the OB. The latter exhibits an input-dependent response, showing a more pronounced increase in odorants that normally elicitgreater responses.
So far, GABAergic terminals are known to terminate exclusively onto inhibitory neurons: GCs (NunezParra et al., 2013) and PGs (Villar et al., 2021), which would disinhibit MC and increase odor-evoked activity. Their findings with respect to basal MCs are, however, counterintuitive. Interestingly, dSACs cells are also known to receive GABAergic inputs from the BF (Sanz Diez et al., 2019). Furthermore, dSACs are a heterogeneous interneuron population targeting mainly PGs and GCs, thus inhibiting bulbar inhibition (Eyre et al., 2008). Inhibition of dSACs will result in an increase of PG and GC inhibition onto MCs. Therefore, inhibition of dSACs could mediate MC spontaneous firing reduction, while direct BF inhibition onto GCs and PGs could underpin an odor-evoked increase in MC activity.
In addition, when Böhm and collaborators evaluated the role of optogenetic GABA release onto MCs activity considering the sniff phase, they found that MCs exhibited an increase in activity during the preferred sniff phase and a reduction of MC’s activity outside of it. The authors proposed that this bimodal MC response could evoke attention-related filtering of sensory stimulation as a means to focus on relevant sensory input instead of irrelevant background stimulus (Böhm et al., 2020). This statement agrees with behavioral data showing the role GABAergic modulation plays in fine olfactory discrimination (Nunez-Parra et al., 2013), although more research is needed to confirm this idea.
GABAergic projections have also been shown to play a role in the modulation of neurogenesis in the olfactory bulb (Hanson et al., 2020). The OB is one of the few regions in the brain that receives a continuous supply of inhibitory neurons throughout life, a process known as adult neurogenesis (Altman & Das, 1965; Lois & Alvarez-Buylla, 1993, 1994). Most of the newly born cells arriving into the OB become GCs and only 5% PGs (Petreanu & Alvarez-Buylla, 2002). Adult neurogenesis allows the bulbar circuit to remain plastic and to be able to modify the inhibition of MCs by the addition of new neurons. Newborn neurons originate in the subventricular zone of the brain, from where they migrate to the OB and become functionally integrated within the OB network. However, more than 50% of the interneurons reaching the bulb die before they are successfully incorporated into the GC and GL layer, suggesting that integration is a highly regulated process (Lim & Alvarez-Buylla, 2016; Petreanu & Alvarez-Buylla, 2002). Indeed, in the OB, olfactory deprivation leads to decreased survival of adult-born neurons (Yamaguchi & Mori, 2005), while olfactory enrichment and learning lead to increased survival, maturation, and integration (Alonso et al., 2006; Lledo & Valley, 2016; Mandairon et al., 2006; Quast et al., 2017).
By using viral labeling techniques to map neuronal circuits on mice brain slices, it was shown that GABAergic projections from the HDB synapse onto immature adult-born GCs in the OB. In addition, through virusmediated GABAergic inhibition, it was shown that these synapses promote the survival of these adult-born neurons (Hanson et al., 2020). Since adult-born GCs had been found to play a critical role in fine olfactory discrimination (Alonso et al., 2012), the Hansen and collaborators result represents a source of long-range input modulating plasticity in the adult OB and a close relationship between learning, circuit maintenance, and GC function, supporting the importance of inhibitory cells in olfactory-mediated behaviors.
6. The Role of BF Projections in the Piriform Cortex
The role of BF modulation in the olfactory cortex had been less studied although some research had been performed in the piriform cortex. Since the OB and piriform cortex is only one synapse away, it is believed that changes in OB output will have a strong impact on pyramidal neuronal activity. For example, it has been shown that the sparseness of the MCs responses is directly correlated with pyramidal neuron sparseness (Apicella et al., 2010). In other words, when a small number of MCs respond to an olfactory stimulus, a smaller number of pyramidal neurons will be involved in creating the representation of a precept. As mentioned above, a sparse code could benefit precept discrimination. Despite the relevance of bulbar output in olfactory processing, how cholinergic-regulated inputs from the OB affect pyramidal neuronal dynamics in vivo the piriform cortex remains largely unexplored (Luskin & Price, 1982). Computational modeling has suggested that Ach increases synchronization and sparseness of the bulbar input to the piriform cortex, promoting more stable learned representations in the piriform cortex (de Almeida et al., 2013; Devore et al., 2014).
Similarly, the PC itself receives cholinergic innervation from the BF (Saper, 1984) where muscarinic receptors can be found (Constanti & Sim, 1987; Saar et al., 2007; Thomas & Westrum, 1989). Studies performed in brain slices have suggested that in this region Ach increases pyramidal neuron excitability (Hasselmo & Bower, 1992). It is believed that pyramidal neurons translate the spatiotemporal inputs from the OB, transforming them into a rate code (changes in firing rate; Gire et al., 2013; Miura et al., 2012). Thus, changes in the excitability of pyramidal neurons and their ability to fire action potentials will have an impact on the generation of olfactory representations. In fact, electrical stimulation of the HDB/MCPO milliseconds before the MC layer was electrically stimulated produces a profound inhibition in the piriform cortex of anesthetized rats (Rosin et al., 1999). Additionally, acetylcholine release due to attentional processes and learning can also cause persistent modifications in pyramidal cell activity (Fletcher & Chen, 2010), such as through long-term potentiation (Hasselmo & Barkai, 1995; Patil et al., 1998), or reduced spike afterhyperpolarization (Saar et al., 2001). Further functional studies on the role of BF projections onto the piriform cortex in vivo are required to fully understand the role of the BF in olfactory processing.
The possible effects on other elements of the olfactory cortex (i.e., olfactory tubercle, anterior olfactory cortex, and entorhinal cortex) remain unexplored. While BF projections into the entorhinal cortex are well established (Kondo & Zaborszky, 2016; Manns et al., 2001; Saper, 1984), functional analyses remain elusive. The olfactory tubercle is particularly interesting, as it is in close physical proximity to the BF, muscarinic receptors have been described in this region (Caulfield, 1993) and it is functionally overlapped with motivation and reward-seeking states during olfactory mediated behaviors (Gadziola et al., 2020; Wesson & Wilson, 2011).
7. Real-Time Basal Forebrain Activity in Awake Behaving Animals during Olfaction
The effect of BF activity on olfactory tasks has been further dissected, pairing it with electrophysiological recordings in awake-behaving animals. Using electrodes implanted on the HDB in the basal forebrain of rats, Devore and collaborators revealed groups of neurons with different activity patterns during a spontaneous odor investigation and a two-alternative forced choice task (2016). The latter consisted in the repeated presentation of odorants, one of which is linked to a reward, in order to establish olfactory associations. For a precise electrophysiological analysis, the task was divided into three epochs: a baseline epoch before the trial begins, an initiation epoch that starts when the animal was given access to the odorants, and an investigation epoch when the animal approaches the odorant ports. When the rewarddriven behavioral task was compared to the spontaneous investigation of an odorant, they found that the baseline rates of HDB neurons were higher and that more neurons were engaged in the rewarded task compared to spontaneous investigation. A closer look into the behavioral epochs revealed two types of HDB neuronal temporal dynamics: one fast and transient and another that was slower or tonic. This was found during trial initiation and odor investigation, where neurons could either increase or decrease their activity for milliseconds to seconds. Interestingly, as the animals became proficient in the task, this response to task demands diminished, suggesting that it is highly relevant to the olfactory learning process (Devore et al., 2016).
Further characterization of HDB neurons through tetrode recordings in self-initiated olfactory go/no go tasks shows how neurons from the BF are involved in different behavioral epochs (Nunez-Parra et al., 2020). The go/no go task involved a test chamber, in which the animals could voluntarily enter an odor port and be presented with one of two odorants (rewarded and unrewarded). Thirsty animals are trained to lick a water port in response to a rewarded odorant -and get water as a reward- and to refrain from licking in response to the unrewarded odorant. It is important to notice that in a go/no go task, animals are trained to refrain from performing an action in response to an odorant, as opposed to the two-alternative forced choice where they have to act (choose a port) after sniffing the olfactory cue. This implies that the two tasks could recruit different neuronal circuits or neuronal circuits with distinct dynamics, as might be the case of the basal forebrain in particular. In the go/no go task, three behavioral epochs were determined: an initiation epoch before the animal entered the odor port, an odor epoch initiated by the presentation of the odorant, and a reward epoch marked by reward delivery. As a control, some animals were subjected to go/go tasks, in which licking in response to any odorant would get rewarded, so no sensory discrimination is required. It was found that certain neuronal units responded differentially (increasing or decreasing firing rate) to each of the epochs and were not responsive to the go/go task. Interestingly, BF neurons were recruited during the trial initiation epoch and even some milliseconds before, possibly relating to anticipatory behavior. Anticipatory behavior had been proposed to be necessary to predict future outcomes, improve sensory perception and performance (Bastiaansen & Brunia, 2001; Jaramillo & Zador, 2011; Nobre et al., 2007). Furthermore, these patterns of activity became more apparent as the animals improve in the task, that is, the number of BF neurons recruited on each epoch increases as the animal learns to associate the odors with the reward. Animals that were evaluated selectively expressed ChR2 in cholinergic neurons and in addition to a tetrode implant, an optic fiber was implanted in the basal forebrain. Once the animal finished each session, a light protocol was applied to identify through optotagging which neurons were, in fact, cholinergic. Cholinergic units, as identified by their response latency to the light stimulus, were recruited during odor and reward delivery. Cholinergic units lacked the response to the reward, indicating that BF neurons recruited during this epoch were noncholinergic neurons (Nunez-Parra et al., 2020).
As we mentioned before, the BF is a complex neuronal network with a heterogeneous neuronal population not only projecting massively to the brain but also regulating neuronal activity locally within the region. Indeed, Ach levels vary in the BF during an olfactory-mediated task (Hanson et al., 2021). Analyses using fiber photometry within the BF showed dynamic and bidirectional variation in cholinergic tone during olfactory discrimination go/no go tasks. These experiments measured the fluorescence emitted by the exogenously expressed indicator proteins GAch2.0 (sensitive to acetylcholine) through a fiber optic implant in the HDB in freely moving mice. Again, this go/no go task was segmented into three epochs: during odor presentation, between odor presentation and reward seeking, and after reward delivery. The cholinergic tone in the BF fluctuated throughout the epochs, rising during odor presentation, but exhibiting a notable increase during reward seeking behavior with a consequent drop after reward delivery. This latter effect was not apparent in go/go trials, suggesting that post-reward suppression is dependent on the task context and the association between odorant and reward. Surprisingly, this pattern showed no difference as the animals became proficient in the task. The difference between this result and the one presented by Nunez-Parra et al. (2020) could arise from the fact that the latter evaluated the very first training session animals associated an odor with the reward, while Hanson and collaborators (2021) chose trials with slow learning rates that could occur after initial training. Moreover, Nunez-Parra et al. studied both cholinergic and GABAergic neuronal activity in the BF, so the main effect they observed could be mediated by GABAergic neurons.
To further study the BF dynamics during a go/no go task, Hanson and collaborators used mice expressing the calcium indicator GCaMP6M in BF GABAergic neurons and recorded their fluorescence emission through the epochs (2021). GABAergic neuron activity rose with the odorant presentation, even when the unrewarded odorant was presented, and started dropping before reward delivery, suggesting that the GABAergic neuron activity profile was related to odor detection and not to reward expectation as the cholinergic tone. However, the decrease in GABAergic neuron activity was steeper after reward delivery, which the authors attributed possibly to the decrease in the BF cholinergic tone (Hanson et al., 2021).
Taken together, these investigations through different experimental designs have helped to partially unveil the role of top-down BF control in olfactory-mediated behaviors in vivo. The results show that the BF cholinergic and GABAergic circuits regulate the activity of neurons in the olfactory bulb differentially and bidirectionally depending on the environmental context. Specifically, on the one hand, acetylcholine released in the glomerular layer regulates the MCs cell apical excitability in an odor concentration dependent manner, while spontaneous and odor evoked spiking activity is enhanced, sharpening MC response. On the other hand, GABA release through inhibitory neurons suppresses MC spontaneous and enhances MC evoked responses. Locally within the BF, cholinergic and GABAergic circuits regulate each other during olfactory mediated behavior adding additional complexity to the BF top-down modulation. Notaby, the activity in the basal forebrain of behaving animals fulfills a crucial role in olfactory processing, integrating relevant information, such as attention, experience, anticipation, and reward expectations, to prime the neurons in the olfactory bulb for appropriate information intake and olfactory discrimination (Böhm et al., 2020; Devore et al., 2014, 2016; Hanson et al., 2021; Ma & Luo, 2012; NunezParra et al., 2013, 2020). In this way, we suggest that the basal forebrain may be acting as a coordination center for sensory processing and adequate decision-making, yet several questions remain unanswered.
For instance, how BF could regulate the temporal dynamics in the OB in vivo has not been studied. Computational modeling has suggested that cholinergic modulation is likely to be responsible for the generation of gamma oscillations (Li & Cleland, 2013), while in vitro experiments have found that GABAergic afferents activation decreases the power of evoked theta oscillation in the glomerular layer and gamma oscillations recorded in the granular layer (Villar et al., 2021). On the other hand, there is evidence showing that BF cholinergic neurons are heterogeneous and that particular subpopulations could innervate different neuronal types in the bulb (Case et al., 2017). More research evaluating the role different cholinergic projection neurons could have in olfactory discrimination would be valuable. Finally, one group found that optogenetic stimulation of BF cholinergic neurons during a passive discrimination task with prolonged odor presentation, increased odor salience, and investigation time to previously habituated odors (Ogg et al., 2018). Yet, more direct and dynamic modulation of the cholinergic and GABAergic circuits during behavior, for instance through optogenetic control of neuronal activity during operant conditioning tasks (e.g., go/no go), would help to expand the understanding of the role the top-modulation from this region plays in olfactory perception and decision-making optimization.
8. Basal Forebrain in Autism Spectrum Disorder
The BF plays such a relevant role that any alterations in its neuronal activity could profoundly alter sensory, cognitive, and ultimately behavioral processes. Individuals with neurodevelopmental disorders, such as autism spectrum disorder (ASD), exhibit deficits in social communication and interaction, restricted interests and repetitive behaviors (Hodges et al., 2020; Lam et al., 2008), atypical attention (Chien et al., 2015; Murphy et al., 2014), and impairment in executive functions (Bangerter et al., 2017). Notably, ASD patients also exhibit alteration in sensory perception in the visual (Chung & Son, 2020; Shah & Frith, 1983; Stevenson et al., 2019), auditory (Yu & Wang, 2021), gustatory (Boudjarane et al., 2017), tactile (Cascio et al., 2015; Foss-Feig et al., 2012), and olfactory modalities (Ashwin et al., 2014; Bennetto et al., 2007; Boudjarane et al., 2017; Dudova et al., 2011; Suzuki et al., 2003). Interestingly, recent evidence has pointed out that most of the abnormal social behavior and cognitive function in ASD might be secondary to inappropriate filtering of daily life sensory stimuli (Robertson & Baron-Cohen, 2017). In fact, the latest international diagnostic criteria for ASD now includes sensory alterations as a core diagnostic feature. These are present very early in development, even before other symptoms are evident (American Psychiatric Association, 2013; Robertson & Baron-Cohen, 2017). The consistent phenotype of sensory alterations and olfactory hyper and hypo-sensitivity, in particular in neurological diseases, indicate that olfaction could be an important key to study specific disease mechanisms. Specifically, differences in olfactory discrimination, detection and pleasantness had been found in most but not all studies (LyonsWarren et al., 2021). Functionally, in the olfactory system of adults with ASD, through functional magnetic resonance imaging, less activation of the piriform cortex in odor identification tasks has been observed (Koehler et al., 2018). Differences in olfactory-event related potential measured through electroencephalograms had also been detected (Okumura et al., 2020). The anatomical and physiological alterations underpinning olfactory deficiencies are not known and it is unclear if these sensory impairments are associated with anomalies in the neuromodulation of BF, despite the evidence showing anatomical and functional differences in the BF of ASD patients. For instance, researchers have found that ASD patients exhibit abnormally small neurons in the septal nucleus of the basal forebrain (Bauman & Kemper, 1985; Kemper & Bauman, 1998), while in the vertical limb of the diagonal band of ASD children, unusually large neurons had been found (Kemper & Bauman, 1998). In the same region, a decreased number of neurons have been found in ASD adult men with intellectual disability (Bauman & Kemper, 1985). Finally, in post-mortem studies of autistic children, the neurons of the magnocellular preoptic nucleus showed a smaller size than the control group (Wegiel et al., 2014) and diminished gray matter in the BF (Riva et al., 2011). Alterations in acetylcholinesterase activity levels, an enzyme that degrades acetylcholine, have also been found in individuals with ASD. Specifically, the fusiform gyrus, a region that is innervated by the BF and is activated during face viewing, showed lower acetylcholinesterase activity. Interestingly, a negative correlation between acetylcholinesterase activity and social disability in autistic adults was found in the same study (Suzuki et al., 2011). However, another study did not find significant differences in the activity of acetylcholinesterase and acetyltransferase in the cortex and BF of ADS adults (Perry et al., 2001). Differences in the concentration of choline, a molecule required for Ach synthesis, have also been found in individuals with ASD. In the prefrontal cortex, an area also heavily innervated by the BF, choline levels were higher in adults with Asperger syndrome, levels that were correlated with the degree of social impairment the participants had (Murphy et al., 2002). Conversely, Friedman and collaborators observed less choline in the left thalamus, the right superior temporal gyrus, and the right medial and temporal lobe of autistic children (2003), along with decreased gray matter concentrations of choline (Friedman et al., 2006). This data, altogether, suggests abnormal acetylcholine release and degradation in autistic individuals.
Another aspect of cholinergic function that has been associated with ASD is the expression of acetylcholine receptors. Several studies have associated nicotinic receptor mutations with autism (Bacchelli et al., 2015; Chilian et al., 2013; Friedman et al., 2003; Koevoet et al., 2021; Lowther et al., 2015; Oikonomakis et al., 2016; Suzuki et al., 2011). Also, the amount of cortical M1 receptors was shown to be 30% lower than normal in autistic adults with mental retardation, with significant differences in the parietal cortex (Perry et al., 2001). Moreover, the immunochemical analysis indicated lower levels of both the α4 and β2 nicotinic receptor subunits in this region (Martin-Ruiz et al., 2004; Perry et al., 2001), suggesting a lower number of nicotinic receptors overall.
Importantly, despite the poor understanding of abnormalities of the cholinergic system in autism, encouraging results have already been obtained by testing cholinergic drugs in ASD. Several autistic symptoms can be improved with cholinergic drugs, such as irritability (Ghaleiha et al., 2014; Hardan & Handen, 2002; Lewis et al., 2018), social interaction deficits (Ghaleiha et al., 2014), social communication (Chez et al., 2004), atypical attention (Olincy et al., 2016), and repetitive behaviors (Karvat & Kimchi, 2014; Wang et al., 2015). Interestingly a novel therapy that has been used in ASD patients is the electrical stimulation of the vagus nerve. It has been found that this treatment improves the mood and social interaction of autistic patients (van Hoorn et al., 2019), but only recently it has been shown that the stimulation of the vagal nerve is mediated by BF cholinergic signaling (Bowles et al., 2022).
As mentioned before, the mouse olfactory system is a notable experimental model to study BF neuromodulation and olfactory processing. Biomedical research using transgenic mice exhibiting analog symptoms as ASD individuals, such as the Fmr1 -/or Tbr1 +/(Bodaleo et al., 2019; Ergaz et al., 2016; Lyons-Warren et al., 2021), could give insights into the role the BF could play in sensory processing alterations. Moreover, how sensory deficits trigger other symptoms observed in ASD individuals still needs to be tested. In humans and other mammals, the olfactory system is closely linked to emotional responses that can range from extreme pleasantness to extreme disgust and aversion (Slotnick & Weiler, 2009). Hence, due to the close anatomical relationship between the brain regions processing olfactory information and emotions, such as the amygdala (Soudry et al., 2011), research studying the BF role in olfaction in transgenic animals could add further information on the social interaction deficits exhibited by ASD individuals and the neurobiological basis of the syndrome.
9. Conclusions
Sensory processing heavily relies on the appropriate integration of bottom-up and top-down information. The BF plays a fundamental role modulating and integrating the activity of several sensory processing regions. The latest advances in neuroscience research allowed investigators to start shedding light on the specific role of the BF neuronal pathways involved in the generation of adequate sensory representation, particularly in olfaction. It is recruited and necessary for olfactory mediated behaviors, including fine odor discrimination, attention, and reward association. Optogenetic, electrophysiological, and behavioral approaches have been revealing, but some elements remain underrepresented. The complexity of the BF circuitry, the heterogeneity of OB innervation, and the differential cholinergic and GABAergic receptor subtypes expression have made it difficult to clarify and isolate the role of the BF system in olfactory information processing. Some consensus throughout the research shows that the cholinergic and GABAergic systems act in a complementary way to increase neuronal gain and signal-to-noise ratio of the olfactory bulb, sharpening MC olfactory representations. The cholinergic and GABAergic centrifugal pathways are intimately coupled to mediate olfactory-mediated behaviors, thus caution has to be taken when the effect of them is independently evaluated. To continue developing the perspective of the basal forebrain as a center for sensory modulation and decision-making, we find it necessary to further expand research into the piriform cortex, a region that is essential for odor-object perception and largely unexplored. The role the BF plays regulating the temporal dynamics in the OB is also completely missing in vivo. Further investigation is needed to dissect the role of BF and other nuclei (e.g., VDB or VP) play in olfactory mediated behavior to establish a link between the basal forebrain as a center for signal integration and sensory decision-making.
Finally, great efforts are being made to understand the neurobiological alterations in ASD. Up to date, only treatments and pharmacology managing some of the symptoms are being used. It is imperative to find other approaches and hypotheses to move the field forward. In that line, alterations in the BF of individuals with ASD are becoming evident, but no studies have explored its role as a sensory neuromodulator underpinning the hyper or hypo sensitivities across sensory modalities found in ASD patients. This is relevant since sensory alterations could mediate some of the other symptoms found in these patients such as gaze avoidance, social interaction deficits, and irritability.
Thus, research using animal models of ASD to study the relationship between BF and sensory processing is a welcome window of opportunity.