1. Introduction
Due to the rapid increase in world population during the recent years, industrial and technological development in many different areas such as agriculture, health, transportation and engineering has accelerated. However, the growing need for food, materials and energy has not made many considerations for sustainability, since a large part of the supplies come from non-renewable sources [1]. In particular, the use of fossil fuels has had an environmental impact on a global scale due to the production of greenhouse gases. [2]. From 1850 to present, the atmospheric concentration of CO2 has increased from 270 ppm to almost 400 ppm and it is expected that by the end of this century it will increase up to 700 ppm [3]. This represents a global concern with respect to ecological, geopolitical and social impacts.
Biotechnological processes offer the opportunity to redesign traditional industrial practices through implementation of new and more sustainable approaches [4-7]. For instance, fermentation technology was key to the development of first and second generation biofuels (i.e. bioethanol), which are view worldwide as possible renewable energy sources [8]. Despite the obvious advantages associated with biotechnological processes, some of the fundamental global challenges still require additional efforts. One of the most representative and influential research lines developed during the last decades is microalgae biotechnology. These photosynthetic microorganisms are able to transform sunlight, CO2, inorganic nutrients and water into ingredients to produce food, feed, fertilizers, biofuels, as well as other products. [9]. In these ways, microalgae can help to reduce the atmospheric concentration of greenhouse gases, without compromising food and energy security. Although many technically feasible bioenergy processes can be developed from microalgae today, much work must be done before this approach becomes an economically viable and competitive with an oil-based economy [10].
In that sense, the application of the concept of biorefinery as a platform for the production of microalgal biomolecules has recently been proposed. This process involves different serial operations designed to produce biomass rich in products that can then be extracted, including high-value molecules such as nutraceuticals, biomaterials, and lower value / large market compounds such as ethanol, biodiesel or methanol. [11,12]. Fig. 1 illustrates the ideal biorefinery process based on microalgae.
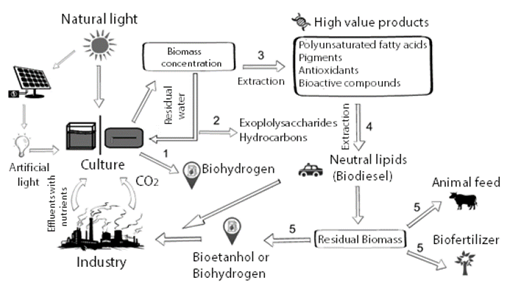
Figure 1 Proposed microalgae cultivation and utilization process including a number of products that could be obtained under the concept of biorefinery.
The biorefinery concept is relatively new, as it was first mentioned as a "green biorefinery" in Germany in 1977 [13]. Basically, this concept is homologous to the well-known oil refinery in which crude oil is fractionated and transformed into a wide spectrum of products [14]. The biorefining process uses similar operations to those used in oil refineries, such as distillation, thermochemical conversion and separation of products, through an integrated process of fractionation and conversion of its components. In addition, similar products are generated in terms of chemical properties and final applications [15]. However, a biorefinery also offers the opportunity to deploy sustainable processes considering biomass as a renewable raw material, since it comes directly or indirectly from photosynthetic products or industrial waste rich in organic matter. [16].
With this review, we aim to highlight some of the most promising alternatives to take advantage of the photo-biochemical reactions carried out by microalgae, from the simplest molecules to more complex and valuable molecules. It also explores some of the emerging and developing technologies that are being applied to overcome the current technical and economic barriers of this industry.
2. Low complexity products derived from microalgae
2.1. Biofuels
The increase in energy demand and advances in biofuel production have drawn increased interest into studies on the use of microalgae as potential sources of third generation biofuels [20].
Considering the state of the art of microalgae technology and today oil prices, algae cannot currently be a considered representative alternative for fossil fuels in the near future [21]. One of the most relevant bottlenecks is the high cost of infrastructure investment and downstream operations such as concentration, dehydration, transformation and purification. The cost of production for the oil and biodiesel from microalgae is estimated to be USD 3.46 / L and USD 3.69 / L respectively. [22]. By contrast, biodiesel obtained from other crops is less expensive such as soybean biodiesel (USD 0.57 / L - USD 0.67 / L), Fischer-Tropsch biofuels from corn fiber (USD 1.13 / L - USD 1.28 / L) and rapid pyrolysis biodiesel from wood chips (USD 0.58 / L) [22,23].
As a result, several efforts have been made to develop faster and cheaper transformation processes. These technologies are mainly focused on optimization of energy and nutrient utilization efficiency, as well as modification of the cellular characteristics and growth conditions to simplify downstream operations. Although some traditional technologies are well known today, we will briefly describe some of the new approaches that are currently under development.
2.1.1. Biodiesel
Biodiesel is traditionally produced by the transesterification of triglycerides, from a renewable raw material, which react with a short-chain alcohol in the presence of a basic or acid catalyst, yielding alkyl esters of fatty acids biodiesel [17]. Currently, lipids from soy, rapeseed and African palm are the main source for their production. However, biodiesel from these raw materials has had many ethical objections due to its competition with food production, which causes fluctuations in food prices and jeopardizes food security [24].
Microalgae species such as Desmodesmus sp., Monoraphidium sp., Chlorella sp., Nannochloropsis sp. show lipid accumulation capacities of 20-40%, exhibiting rapid growth and high biomass production, which implies higher lipid productivity, and thus considered promising candidate strains for industrial biodiesel production. It is estimated that with microalgae capable of accumulating 30% of lipids, 53 t / ha of lipids could be obtained annually, which higher than obtained from palm oil (3.62 t / ha) or soy (0.4 t/ ha) [24].
Conventional biodiesel production from microalgae consists of concentrating the biomass in suspension, followed by drying, cell wall disruption and lipid extraction. Subsequently, the lipids are transformed into biodiesel by trans-esterification [25]. However, the most energy intensive operation is biomass dehydration [26], and for this reason, some authors have proposed methods for direct transformation of intracellular lipids to biodiesel through processing of wet biomass [27-29]. Another common issue related to microalgal biodiesel is the presence of free fatty acids. These molecules are converted into soaps when they react with alcohols and alkaline catalysts such as potassium hydroxide during transesterification, so the use of acid catalysts such as sulfuric acid has been proposed. [30-32].
This problem is also being addressed by the use of new heterogeneous catalysts that lead to the direct conversion of free fatty acids into biodiesel [33]. Gulde et al. (2017) evaluated a tungsten / zirconium heterogeneous acid catalyst (WO3 / ZrO2) to transform lipids from Scenedesmus obliquus into biodiesel. The catalytic efficiency of (WO3/ZrO2) was compared with a homogeneous acid catalyst and an enzymatic catalyst in terms of conversion efficiency, reaction parameters, energy consumption and reuse. In their experiments, the zirconium catalyst showed a maximum conversion to biodiesel of 94.58%, comparable with the conversion of biodiesel from a homogeneous catalyst and a higher conversion than the enzymatic catalyst. The time required for the heterogeneous catalyst was shorter, while the energy consumption was higher among the selected catalysts [34]. In other studies, Cheng et al. (2016) used graphene oxide (GO) as a solid acid catalyst to transform lipids extracted from wet microalgae into fatty acid methyl esters (FAMEs), through acid-catalyzed transesterification (groups SO3H) in GO catalysts. The efficiency of lipid conversion to FAME was 95.1% in microwave-assisted transesterification reactions with GO catalyst at 5% by weight at 90 ° C for 40 min [35].
In situ trans-esterification is another alternative to produce biodiesel starting from wet biomass [36]. It has been demonstrated that the average energy consumption for drying and transesterification is 5.42 MJ / L of biodiesel, but being a process based on hydrolysis and esterification of wet biomass, it has a theoretical energy consumption of 1.81 MJ / L [37]. On the other hand, the enzymatic conversion has also been evaluated as an efficient method of biodiesel production. Tian et al. (2016) evaluated a combination of lipase (NS81006 and Novozym435) in a two-stage process, finding a yield of FAMEs of 95% [38]. Kim et al. (2016) used Aurantiochytrium sp. KRS101 for enzymatic trans-esterification due to the simplicity of cell disruption associated with its thin cell wall, but this case is an exception because most microalgae present a very resistant cell wall [39].
Normally, microalgae store the lipids intracellularly, and thus it is necessary to both disrupt the cell walls and lyse protoplasts release these molecules. However, such a process is destructive, which implies an irreversible loss of the biological catalyst. Some authors have investigated a novel methodology known as “Milking" [40-43]. With this method, cells are exposed to biocompatible solvents that can leach intracellular lipids without compromising cell viability [44]. Atta et al. (2016) evaluated an integrated process of production and extraction of lipids of Chlorella vulgaris using dodecane and tetradecane as biocompatible solvents in a biphasic system. They found that C. vulgaris survives up to four cycles of in situ extraction. The highest amount of lipids (1175 mg / l) in the solvent phase was achieved with a maximum lipid recovery ratio of 47 ± 5% during the first cycle when a concentration of 20% dodecane was used as a solvent [45]. They also concluded that the lipids extracted with this system are suitable for the production of biodiesel. Griehl et al. (2014) proposed a continuous extraction system for Botryococcus braunii SAG 807-1. The authors developed a method to cultivate the cells and extract hydrocarbons simultaneously, avoiding the operations of high energy demand for filtration, dehydration and cell breakdown [41].
2.1.2. Hydrocarbons
Hydrocarbons are very stable compounds and a substantial source of energy in the world, most of these are naturally found as petroleum [46,47]. However, some studies have shown that cyanobacteria, red algae and dinoflagellates produce noticeable level of hydrocarbons, finding quantities close to 6% of their dry weight. [48-52]. Of particular note, green algae have been shown to have potential for the production of hydrocarbons [50], especially the microalga Botryococcus braunii, which can contain up to 86% of hydrocarbons in dry weight basis [53]. B braunii has the capacity to produce bio-hydrocarbons similar to petroleum, capable of being transformed into high octane gasoline through hydrocracking processes [54,55]. This strain is classified into three different races according to the type of hydrocarbon it produces. Race A produces odd carbon chains (C23-C33) n-alkadienes (mainly diene and triene hydrocarbons); race B produces triterpenoid hydrocarbons, such as botryococenes (C30-C37) and methylated squalene (C31-C34), while race L produces licopadiene which is a C40 tetraterpene [56].
Diesel, gasoline and jet fuel are mainly composed of C4-C23 alkanes due to their high energy content and hydrophobicity. One of the approaches to producing useful hydrocarbons from microalgae is based on the isoprene molecule. This short-chain volatile hydrocarbon is derived from the first products of the Calvin cycle of photosynthesis, and is produced at substantial rates under certain conditions of environmental stress [57]. The heterologous expression of isoprene synthase in combination with the enzymes of the mevalonic acid pathway has improved the isoprene yield of approximately 2.5 times, compared to that measured in the cyanobacteria transformed only with the isoprene synthase gene. [58].
Another way analyzed by some authors to increase hydrocarbon production is to induce microalgae to produce ethylene. Sakai et al. (1997) studied the conversion of CO2 to ethylene using Synechococcus PCC7942 expressing the ethylene-forming enzyme (EFE) of Pseudomonas Syringae phaseolicola PK2. This work showed that this recombinant cyanobacterium was capable of producing ethylene and 5% of the total carbon incorporated by the cell was transformed into this hydrocarbon.
Regarding the practical evaluations of the fuel obtained from microalgae, there are limited examples that can be mentioned. The company Solazyme has successfully tested the fuel derived from microalgae in real flights. Continental Airlines flew a plane using a fuel mixture with 40% bio-jet microalgae. In addition, the United States Navy tested a mixture with 50% microalgal fuel in a helicopter [59].
2.1.3. Ethanol
Biofuel production from microalgae has been focused on biodiesel, but increasing interest exists for approaches involving bioethanol and biohydrogen as well [20]. This is a feasible technological development because microalgae can reach up to 50% of their dry weight in carbohydrates (glycogen, cellulose and starch), which can then be transformed to ethanol by fermentation [60]. The production of bioethanol is determined by the biochemical composition of the biomass and the biodegradability of the cell wall. Unlike plants, these microorganisms do not produce lignin, which is a recalcitrant aromatic polymer that residue utilization difficult, thus better conversion performance is expected with lignin-free algal biomass [12,61].
Conventional ethanol production is an energy-demanding process due to the multiple steps required, including pretreatment, the cost of enzymes for hydrolysis and bacteria or yeasts for fermentation, in addition to the costs associated with separation and purification [62]. The improvements related to this route are mainly focused on biomass production, hydrolysis and fermentation efficiency. Some authors have reached efficiencies of saccharification from 80% to 98% and a transformation to ethanol of up to 80% [63-65].
Although the transformation of biomass from microalgae to ethanol is usually done by hydrolysis and fermentation, there are new processes in development such as dark fermentation and photofermentation using transgenic strains. Dark fermentation is an alternative method proposed to produce ethanol using microalgae. Basically, the cells are autotrophically grown to accumulate reserve compounds and fermentative metabolism is subsequently redirected to ethanol production by degradation of the intracellular carbohydrate and lipid reserves in the absence of light. This process is still under development with the aim of improving its efficiency [66].
Recently, photofermentation has received special attention because it involves genetically modified organisms and seems to be a promising alternative in terms of efficiency [20]. The cyanobacteria are genetically modified to express the metabolic pathways necessary to convert the photosynthetically-produced compounds into ethanol. In general, microalgae can ferment efficiently, with the additional benefit of using CO2 as a carbon source [67]. Synechocystis sp. PCC 6803 and Synechococcus elongatus sp. PCC 7992 were successfully used to produce ethanol [68], and photofermentation processes have been implemented industrially by Algenol Biotech (USA) [69].
Currently, in addition to advances in fermentation technologies for the production of bioethanol and the accumulation capacity of fermentable molecules such as starch, special efforts have been made to reduce production costs. Some such strategies involve the modification or improvement of cellular processes at the metabolic level to increase the efficiency of the transformation or reduce the cost of the raw material [70].
2.1.4. Hydrogen
Hydrogen is considered an attractive future energy source because of its high energy content per unit mass or energy density [71] and its clean combustion that produces only water as byproduct. The ability of certain microalgae to produce molecular hydrogen was discovered more than 70 years ago [72]. Some studies show the potential biohydrogen production of some microalgae for the, due to the high theoretical efficiency they have for the conversion of light, both in aerobic and anaerobic environments [71,73,74].
There are three types of processes used in the production of biohydrogen: direct biophotolysis, photofermetation (indirect biophotolysis) and dark fermentation; however, only the first two can be used by microalgae, which require a light source to produce hydrogen [75]. Current levels of hydrogen production obtained from wild-type microalgae at laboratory scale vary from 0,04 mLH2 L-1 h-1 [76] to 7,2 mLH2 L-1 h-1 [77]. A known method to increase the hydrogen production capacity is to limit the amount of sulfur. Given this condition the activity of photosystem II gradually decreases and subsequently oxygen release is reduced. Anoxic conditions are favorable for facilitating electron transport with respect to hydrogenase, which gives H2 as a product [78]. The limitation or starvation of other nutrients such as nitrogen and phosphorus can also favor the production of H2, however, the limitation of sulfur seems to be the most influential condition [79]. Although this method can be easily implemented on a laboratory scale, it is not easy to scale if industrial production volumes are considered. Some attempts to carry out this process in tubular reactors have been described but always at working volumes lower than 100 L [80]. To improve the efficiency of this process, alternative approaches have been proposed, such as photobioreactors designed to increase cellular efficiency, integrate the production of microalgae with wastewater treatment, and even genetic engineering. [79]. A parallel transformation scheme is based on the production of microalgae biomass by traditional methods to be subsequently used as a substrate in dark fermentation by other hydrogen-producing microorganisms. (Clostridium, Bacillus, Escherichia, Enterobacter).
Genetic engineering and “omics” are probably the most promising tools to overcome the current bottlenecks of the microalgae energy industry [81]. Chlamydomonas reinhardtii, Botryococcus braunii and Synechococcus elongatus genomes have been completely sequenced, and there are several genetic transformation and analytic tools that convert them into model organisms for hydrogen production [82-85]. In this direction, some work has been done to seek improved photosynthetic efficiency by truncation of photoreceptor antennas. This route leads to a reduction in the activity of photosystem II, decreasing the production of oxygen and thus favoring the activity of the hydrogenase. Parallel to this, the effect of light self-attenuation also decreases [84-86]. To carry out the process on a large scale, some authors have proposed to limit the entry of sulphate to the chloroplast by creating mutant strains with altered activity of the sulphate permease at the chloroplast envelope. [86,87].
2.1.5. Syngas
Among the main thermochemical technologies, biomass gasification is considered another promising process and has the best cost / efficiency ratio for the conversion of biomass into bioenergy, including lignocellulosic biomass [90]. Gasification is a technology that converts carbon-based solid material, such as biomass, into syngas for energy purposes through a thermochemical conversion composed of H2, CO, CO2 and CH4 [91-92]. In the gasification process, the algal biomass is normally heated in deficient quantities under low O2, air or a mixture of both, where the product of the process is an incomplete combustion of the biomass generating synthesis gas, which can improve its yield depending of combustion conditions [93].
In 2014 Duman et al. used residues of Nannochloropsis oculata for gasification in fixed bed reactor at temperatures of 600-850ºC with water steam [94]. The results showed a conversion of carbon of 70% and a syngas composed mainly of H2 (50%) and CO2 (35%), while CH4 and CO were also found in proportions of 10 and 6%, respectively. In 2015 Watanabe et al. performed a catalytic gasification with Ni-Fe/Mg/Al to Botryococcus braunii biomass residues after extracting their lipid material at a temperature of 750 ºC [95]. The results showed a conversion of 91% of the tar formed in the pyrolysis phase into CO, CO2 and CH4 monocarbon gases. The synthesis gas was composed mainly of CO and H2 in a H2 / CO ratio of 2.4. The maximum hydrogen yield obtained in this work was 74.7 mmol g-biomass-1, in which they conclude that it is the highest result up to that moment of those reported in the literature.
2.2. Fertilizers
The use of microalgae for agricultural applications is focused on the in vivo use of cyanobacteria, which have a high ability to fix atmospheric nitrogen (N2) and dispose it in the form of NH3 for the direct assimilation of plants. Although few microalgae have the capacity to fix this nitrogen, their biomass can be used as an ecological input for agricultural crops. In addition, microalgae release growth promoting substances such as auxins and cytokinins, while also solubilizing phosphates, and the inactive biomass provides nutritious organic matter that improves soil fertility and crop quality. The biomass of microalgae can be applied directly, although the previous hydrolysis allows to improve the positive effects of these products on the plants [96]. In flooded crops, especially rice, the positive effect on production attributed to the presence of the freshwater fern Azolla and the cyanobacteria Anabaena azollae that develop a symbiotic relationship is well known. The annual fixation capacity of atmospheric nitrogen of this system is 350 - 670 kg N fixed / ha, about 12 to 20 times higher than the fixing capacity presented by A. azollae isolated [89,90].
Wuang et al. (2016) used the biomass of Spirulina platensis as an agricultural biofertilizer in different types of crops. In this work it was observed that the application of Spirulina biomass caused an increase in the growth (in dry weight) of the plants A. rugula and red spinach in 21.1% and 155.8% respectively, but for Chinese cabbage did not show differences compared to the control [99]. Overall the quality for use as fertilizer depends on the properties of the microalgal biomass and the nutritional requirements of the plant. In order to meet this specific requirement for each plant, the mixture of biomass from different microalga strains has been proposed. This strategy has been useful and effective in achieving the increase in plant growth. In other studies, González et al. (2016) took advantage of the residual water of the microalgae culture, and the cellular extracts obtained after cell disruption and the microalgal biomass was shown to increase germination rates, as well as growth and production of flowers of the tomato plant (Solanum lycopersicum) [100].
3. More valuable molecules obtained from microalgae
3.1. Biomass with nutritional value
Algae and human nutrition have been related for centuries, the first record found in South America dates from 13,000 years ago in Chile, where algae were used as food, and their medicinal use was known [101]. Table 1 illustrates the biochemical characterization of some foods and biomass of different microalgae species. The protein content, carbohydrates and lipids in microalgae depend on each species and the culture conditions. Its comparison with the nutritional value of daily food is favorable in terms of quality, quantity and protein intake. Likewise, the profile of fatty acids present in the microalgal biomass is similar to that found in higher plants, which are essential fatty acids for human consumption [102]
Table 1 Biochemical composition of food for daily consumption and biomass of different strains of microalgae
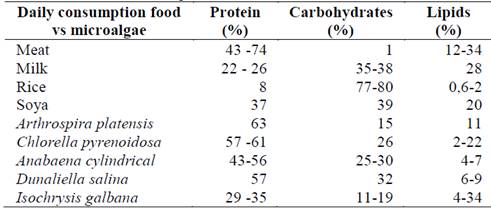
Due to its high protein content, which can exceed 70% in dry weight, vitamin B12, minerals and a high content of ɤ linoleic acid, Arthrospira platensis (Spirulina) is the most commercialized microalgae species cultivated for use as a dietary supplement [103]. The annual production of Spirulina is greater than 5,000 t and the main producers are Japan, USA, India and China [95,96]. In the group of Chloropyceae, Chlorella vulgaris is the most exploited strain due to its high content of protein (50%), vitamins, carotenoids and β-glucan (immunostimulant). The biomass consumption of these microalgae has gained great interest worldwide as food to treat malnutrition. For its therapeutic properties, Spirulina is used for the treatment of cardiovascular diseases, arthritis, anemia and cancer, whereas Chlorella is used to treat problems such as gastric ulcers and neurosis [106].
Currently, there is an emerging market of functional foods from microalgae where a biomass or extracted active substance is added to a food matrix to confer additional nutritional characteristics [107].
Batista et al. carried out various studies on the incorporation of microalgal biomass in different foods such as cookies, pasta and mayonnaise. For example, in one of their investigations, it was observed that the addition Chlorella vulgaris and Haematococcus pluvialis biomass with high carotenoid content increases the oxidative resistance of the mayonnaise lipids and has an emulsifying effect [99,100].
The use of the different microalgal strains as nutritional products is limited by the legal regulations that must be met so that they can be commercialized. Currently, A. platensis and Chlorella sp. are currently the only strains globally allowed to be used as food. However, H. pluvialis and Dunalliella sp. pigments are also produced for use as functional foods. It is expected that in the near future a wide range of strains of microalgae with different nutritional characteristics will be available to expand their use in this field [111].
In animal feed market, especially in aquaculture, the most commonly used strains are Chlorella, Tetraselmis, Isochrysis, Pavlova, Phaeodactylum, Chaetoceros, Nannochloropsis, Skeletonema y Thalassiosira [103,104].
3.2. Pigments
The main role of pigments in microalgae is the light absorption, which is useful for photosynthesis, in addition they can also provide photoprotection against high light intensity. Among the carotenoids produced by microalgae we can find astaxanthin, β-carotene and xanthophylls such as lutein and fucoxanthin. The most common application of natural pigments relies on food industry due to the benefits for human health related to their antioxidant and pro vitamin A properties, and in lesser amounts are used in the pharmaceutical, textile and cosmetic industries [104]. The global market of carotenoid is covered about 50% by astaxanthin and β-carotene.
Haematococcus pluvialis and Dunaliella salina are a worldwide primary source of pigments [107]. H. pluvialis is cultivated in two stages, first under ideal conditions for growth and the second under stressful environmental conditions to induce secondary pigments accumulation [114]. It has been reported that this microalga can produce up to 50 mg / g of astaxanthin under light stress, yielding between 0.5% up to 5% of its dry weight [98,106]. Some authors proposed a single stage process with controlled conditions of nutrient supply, especially nitrogen. However, this process has not yet been carried out on a commercial scale. [107,108]. The price of natural astaxanthin for human consumption exceeds the USD 6.000/kg [17,109].
Similarly, Dunaliella species accumulate high amounts of β carotene, about 10-14% of their dry weight, and have been shown to increase production in response to high salinity and light intensity [105]. Most of Dunaliella cultures are grown in open tanks, taking advantage of their high tolerance to high salt concentrations, which reduces the risks of contamination, and Israel, Australia and the EE. UU are the leading countries in large-scale biotechnological production of β-carotene from microalgae [119].
In relation to lutein, its global market in 2010 was USD 233 million and is likely to increase to USD 308 million by 2018 [120]. Green microalgae produce considerable amounts of this pigment. Chiu et al. (2016) determined that 180 kg of lutein can be obtained annually from 33 tons of biomass / ha of the thermotolerant strains Desmodesmus sp. and Coelastrella sp. grown in open systems [121]. Scenedesmus almeriensis showed a fast growth and high content of lutein for food use and can be produced in closed systems [122], although production in open systems has also been demonstrated [123].
Phycobiloproteins are proteins linked to phycobilins, an accessory photosynthetic pigment, forming a fluorescent active compound present in cyanobacteria and red algae with high potential for application in pharmaceuticals and cosmetics due to its anti-carcinogenic, anti-inflammatory and neuronal protective properties. Phycocyanin (blue) is the main pigment found in Spirulina and phycoerythrin is generally found in rhodophyta Porphyridium. The accumulation of phycobiliproteins in microalgae occurs due to cellular stress generated mainly by light and carbon source availability, in Spirulina platensis the content of phycocyanin varies between 0.11 to 12.7% of the dry weight [124]. These proteins are industrially used as fluorescent marker and in other medical applications [124, 125].
3.3. Polyunsaturated fatty acids
Polyunsaturated fatty acids (PUFAs), especially long-chain fatty acids such as eicosapentaenoic acid (EPA C20:5) and docosahexaenoic acid (DHA C22: 6) are considered essential for humans for being key molecules in health. Due to the low conversion efficiency of the long chains of the omega-3 family, which is about 5% for EPA and less than 0.5% for DHA, whose efficiency decreases even more with age, these fatty acids must be supplemented in the diet [115,116]. Marine microalgae are the primary producers of omega-3 polyunsaturated acids, which are transferred to other organisms through marine food chains. Compared to fish oil, microalgae oil has similar amounts of omega-3, with the advantage of having three times less cholesterol content, has no problems of contamination with heavy metals and toxins, its cultivation is simple and there are some strains that can produce and accumulate a single type of PUFA in a large percentage, facilitating its extraction and purification [117,118]. For example, Crypthecodinium cohnii grows rapidly under heterotrophic conditions, especially when glucose is used as a carbon source and accumulating between 30-50% of DHA dry weight [130].
So far the use Crypthecodinium cohnii and Schizochytrium sp. are the primary sources of commercial DHA which is sold as a supplement in infant formulas [95,120]. Phaeodactylum tricornutum ans Nannochloropsis sp. accumulate EPA, but their commercial production is still not very consolidated. The global market for PUFAs, especially DHA and EPA, is growing exponentially [132]. The consumption of DHA / EPA functional products, especially in regions such as North America, Europe and Asia, represented USD 25.4 billion in 2011 and USD 34.7 billion in 2016 [133]. The price per kg of DHA varies between USD 2,000-3,000, offsetting the high processing costs for the production of microalgal DHA [17].
3.4. Functional polysaccharides
The polysaccharides obtained from microalgae have a strong potential for use in biodegradable polymeric materials or natural bioactive products that have shown benefits for human health such as anticoagulants, antithrombotic, antitumor and immunomodulatory agents [134]. Microalgae can produce a large amount of polysaccharides during their life cycle and secrete them into extracellular medium, and in combination with other biomolecules such as proteins, lipids and nucleic acids, providing the backbone for protective biofilms. These exopolysaccharides (EPS) can be recovered from supernatants after concentration of biomass, without generating any additional waste, constituting another byproduct that can be obtained under the concept of biorefinery [123,124]. The structure, physical and chemical characteristic of said polysaccharides determine their biological activity and final application. In the majority of microalgae species, depending on the culture conditions, the synthesized polysaccharides are heteropolymers composed mainly of a combination of sugars such as glucose, xylose and galactose, among others. [132]. For this reason, there is a broad spectrum of bioactive compounds with different molecular weights, ionic charges and properties that can have a high value application in various industries such as mechanical engineering, food or biomedicine.
Additionally, Chlorella vulgaris and Gyrodinium impudicum have been reported for their ability to generate homopolysaccharides composed of glucose as part of their cell wall and galactose exopolysaccharides, respectively.
4. Sources of microalga biorefinery supplies
The price and availability of the raw materials for the microalgae biomass production represents a significant factor for commercial process, considering that fundamental inputs for its production are carbon, nitrogen, phosphorus and microelements. However, the energy required during incubation, recovery and biomass transformation should also be considered. [125,126].
Bearing in mind the characteristics of the aquatic production system of algae systems coupled to treatment and production to transform industrial wastes into microalgae biomass has raised with the dual purpose of reducing production costs and environmental pollution. The most widely studied alternatives are the capture of CO2 released in combustion processes and the purification of industrial and domestic wastewater. [61,127,128]. Accordingly, we will describe some of the alternatives that are currently under study to provide some of the process inputs in a more cost-effective manner.
4.1. Carbon
Under autotrophic conditions, microalgae use light and inorganic carbon as sources of energy and carbon, respectively. However, some species can grow mixotropically, assimilating organic carbon sources as well, resulting in higher biomass [129,130]. In spite of this last advantage, traditional organic sources (sugars, organic acids, glycerol etc.) turn out to be costly alternatives if they are used in their pure form and could compromise the quality of the open systems due to the proliferation of contaminants [141]. In that sense, the use of inorganic carbon salts, the use of combustion gases and the implementation of systems coupled with nutrient-rich wastewater treatments have been proposed [131,132].
Some authors have evaluated wastewater from pig farms, as low-cost raw materials, as well as effluents from breweries, and by-products and wastes from the food and agroindustrial industry such as cane molasses, silage juice, serum permeate, among others [129,130,133]. These waste streams have been satisfactorily evaluated and are emerging as low-cost raw materials in the preparation of culture media for microalgae [134,135].
4.2. Nitrogen
Nitrogen is the second most important nutrient for microalgae biomass proliferation, its cellular content can vary from 1% to 14% on a dry weight basis [147]. Algae can assimilate inorganic nitrogen molecules such as NO3 -, NO2 -, NO, NH4 + and some cyanobacteria, like Oscillatoria sp., Nostoc sp., Anabaena sp., and some diatoms like Rhizosolenia sp. and Hemiaulusare sp. can fix N2. They can also assimilate N in organic forms, such as urea, peptone, yeast extract, amino acids and purines [136,137]. In urban wastewater, urine provides 70% nitrogen, 40% phosphorus and 60% potassium of the total load and represents approximately 1% of the volume of water [138,139]. This nitrogen/phosphorous rich residue has been used for cultivation of Arthrospira platensis and Chlorella sorokiniana.
Markou et al. (2014) proposed a novel alternative ammonium nitrogen recovery using zeolites as an adsorbent material in wastewater with a high content of nitrogen, dissolved solids, dyes and recalcitrant compounds that hinder the passage of light, which inhibit the growth of microalgae [142]. In a subsequent study, Markou et al. (2015) determined that the microalgae A. platensis and C. vulgaris can grow with the nutrients provided by the ashes of hatchery residues of birds with high content of phosphorus, potassium, and ammonium recovered from the process of drying this residue. Simultaneously, they proposed the use of these microalgae biomass as feed for birds, creating a closed production system [151].
4.3. Phosphorous
Phosphorus is an essential element for different cellular processes like energy storage and transfer in the form of ATP, as well as biosynthesis of nucleic acids, phospholipids, among many other [148]. The content of this element in the algal biomass is generally from 1.0 to 3.3% by dry weight. The traditional sources of phosphorus at the laboratory level are mainly inorganic salts such as K2HPO4, KH2PO4, K3PO4, (NH4)3PO4. Although phosphorus is a minor component of the biomass of microalgae, it is still necessary to identify and evaluate new alternatives for recovery and use of this element, taking into account that for this particular nutrient, there is no a biogeochemical mechanism that recirculate it at the level of the biosphere, in contrast of what happens with carbon and nitrogen [57].
Mukherjee et al. (2015) developed a method of recovering and recycling phosphorus from wastewater generated during rice parboiling using microalgae. They isolated Cyanobacterium sp., Lyngbya sp., Anabaena sp. and Chlorella sp. in eutrophic environments and used them as bioremediation agents. After harvesting the biomass, they used them as a biofertilizer to plant rice seedlings and observed that the release of phosphorus by the action of solubilizing organisms is similar between biofertilizer and chemical fertilizer. [152].
Moed et al. (2015) investigated the use of struvite (MgNH4PO4) as a source of nitrogen, phosphorus and magnesium for the cultivation of C. vulgaris. In the tests they used 721 mg / L of struvite for the Bold Basal Medium (BBM). This modified medium can produce 2.85 g / L of lipids suitable for the production of biodiesel, comparable to the use of BBM without struvite [153].
4.4. Micronutrients
These are elements that appear in very small quantities, on the on the micro-level (μg/L), or parts per billion (ppb). These trace elements act as enzymatic cofactors and are important for the biosynthesis of many cellular compounds. The essential micronutrients for microalgae are mainly Mg, S, Ca, Na, Cl, Fe, Zn, Cu, Mo, Mn, B and Co. Many wastewater and industrial by-products all or a large number of these micronutrients and for this reason they are explored as raw material for the cultivation of microalgae. [131,137].
4.5. Energy requirements for biomass production
Considering the processes of obtaining biofuels derived from microalgae, part of the sustainability of the process lies on the favorable nature of the energy balance [154]. Throughout the process of biomass production and byproducts, a series of operations are involved related to incubation, harvesting, processing and purification [155]. The highest proportion of energy consumption is associated with aeration, pumping, centrifugation, harvesting and drying [156].
Shimako et al. (2016) investigated two bioenergy production systems involving biodiesel and biogas, where electricity and heat are generated. These authors found that the net energy balance was negative for the biodiesel production process. However, the energy balance in the biogas production process was positive, which suggests that this could represent an alternative to establish the process to a greater scale [157]. Doušková et al. (2010), proposed a novel process for the use of organic liquid waste in order to simultaneously produce energy and microalgae. They used vinasse as a substrate for the production of biogas in an anaerobic fermentor and later the biogas was fed to photobioreactors as a source of CO2 for the cultivation of microalgae. Once the biogas is produced, the wastewater from the anaerobic fermentor is processed to obtain an ammonium-based fertilizer that can be used directly as a nitrogen source in the culture medium of the microalgae or for the production of ammonia liquor [158]. Shimako et al. (2016) investigated two bioenergy production systems involving biodiesel and biogas, where electricity and heat are generated. These authors found that the net energy balance was negative for the biodiesel production process, however, the energy balance in the biogas production process was positive, which suggests that this could be the alternative to establish the process to a greater scale [157]. Doušková et al. (2010), proposed a novel process for the use of organic liquid waste in order to simultaneously produce energy and microalgae. They used vinasse as a substrate for the production of biogas in an anaerobic fermentor and later the biogas was fed to photobioreactors as a source of CO2 for the cultivation of microalgae. Once the biogas is produced, the wastewater from the anaerobic fermentor is processed to obtain an ammonia-based fertilizer that can be used directly as a nitrogen source in the culture medium of the microalgae or for the production of ammonia liquor [158].
Sevigné Itoiz et al. (2012), analyzed the life cycle and energy balance in the production of marine microalgae biomass, finding that in closed systems, the total energy requirement was 923 MJ kg-1, whereas for outdoor cultures it was 139 MJ kg-1. Additionally, these authors affirm that during cultivation, the highest energy consumption occurs in the growth stage. Although the energy balance was negative for both systems under the conditions studied, the authors suggest that outdoor cultivation could be optimized to obtain a positive balance considering the use of raceway or flat panel reactors [159].
In addition to the energy required for the operations described above, light energy can also be considered as a critical input concerning the energy balance. The primary source of PAR (Photosynthetic Active Radiation) photons is sunlight, which is free and available throughout the year in some regions. However, sudden variations in irradiation and change in weather and seasonal conditions can be detrimental for production. In contrast, the use of artificial sources such as fluorescent tubes, high intensity discharge lamps (HID) and LED lights, offer continuity and stability in the energy supply but can represent prohibitive costs in terms of investment and operation in massive cultures [154]. Under conditions of artificial lighting, Blanken et al. (2013) found that the energy demand during the process can be compared with the enthalpy of combustion of algae biomass (0.477 MJ C-mol-1). They concluded that a PAR light efficiency of 2 μE s-1 W-1 and a biomass/light yield of 1,0 a 1,5 g-DW E-1, only 4% to 6% of the electric energy supplied initially is conserved in the biomass, so that a large amount of energy is lost in cultures with artificial light and the use of this should be avoided. However, the electrical energy required for the cultivation with artificial light could be generated as 'green' energy by photovoltaic, wind or geothermal [154].
The light quality influences cell growth and biochemistry, so artificial lighting allows manipulation of the final biomass for specific uses. The use of artificial light ensures a better regulation of the flow density of photosynthetic photons, of the photoperiod and the light spectrum in the production of microalgae. LED light can also be used to adjust the biochemical composition of microalgae biomass through individual wavelengths at different intensities or frequencies of light pulses [160]. However, the implementation of artificial lighting feasibility to produce microalgae will be subject to the improvement of the energy efficiency of the sources and their cost of production, as well as the biological improvement of the photosynthetic efficiency.
5. Microalgae biorefinery as an integral system
A biorefinery is defined as a facility which integrates the process of conversion of biomass and suitable equipment to simultaneously produce different products such as fuels, energy and added value chemicals with a minimum of waste and emissions [150,151]. A microalgae platform can easily fit this concept since each species produces a certain amount of lipids, carbohydrates, proteins, among other by-products, so that biomass can be used for different purposes [152,153]. To date, microalgal biorefinery processes in general have concentrated in carrying out the cultivation of microalgae in wastewater for simultaneous water treatment coupled with biomass generation from the microalga-bacteria consortium, which in turn can be allocated to the production of biofuels [165].
With respect to the complete utilization of microalgal biomass, few studies at laboratory scale have been found where different techniques for separating biomass components are evaluated to obtain two or more products in parallel. In general, these investigations point to the use of microalga biomass for lipid extraction and biodiesel production, with the generated residual biomass applied to anaerobic fermentation processes to generate biogas or bioethanol [18]. So far, the number of products obtained under the concept of microalgae biorefinery is up to three to four metabolites, as can be seen in Table 2, which shows a summary of some experimental works that have developed the microalgal biorefinery concept.
Ferreira et al. (2013) proposed an economic, energy and CO2 emission analysis comparing two microalgal biorefinery processes. In the first case, supercritical CO2 was applied for the extraction and fragmentation of lipids and pigments, whereas, in the second case a Soxhlet method is used to obtain lipids. Additionally, residual biomass generated from both extraction process was used for the production of biohydrogen, and these two methodologies were compared with a simple cultivation process for Nannochloropsis sp. biomass generation destined for the generation of H2 [177]. The authors determined that while the extraction by Soxhlet (245 MJ/MJlip prod - 15357 g CO2/MJ lip prod) consumed less energy and produced less CO2 that lipid recovery by supercritical fluids (291 MJ/MJlip prod - 18258 g CO2/MJ lip prod), supercritical CO2 allowed fractionation and obtaining of pigments of high added value that do not contribute to an additional expenditure on energy and bring a higher economic benefit. The coupling of the extraction of lipids and the use of residual biomass for an anaerobic process allows the reduction of energy consumption (214-218 MJ/MJprod) and CO2 emissions (13,982-16,800 g/MJ prod) compared to direct use of complete biomass in the production of biogas (9058 MJ/MJprod - 591,112 g/MJprod). Furthermore, the biorefinery process via supercritical fluid and anaerobic fermentation of the residual biomass has a low production cost of about USD 0.2 / g biomass, which represents approximately half of the cost generated by the biorefinery process via Soxhlet and substantially more economical than the cost of biomass production used only for the production of biogas. Therefore, it is believed that the biorefinery is the only scenario in which the biofuel from microalgae could become economically competitive.
The total, neutral (used for biofuel) and membrane (polyunsaturated fatty acids) lipids were also successfully obtained in the above case studies. Long-chain polyunsaturated fatty acids have a higher price in the pharmaceutical or food market and need to be free of hazardous solvents for such markets, and the extraction of lipids can be done by supercritical fluid with CO2 and subsequently each type of fatty acid can be concentrated using the urea complex method. This method entails trapping the the linear chains of saturated fatty acids inside the urea leaving the PUFAs in either solution or centrifuged with a temperature gradient, taking advantage of the differential property at the freezing points of different fatty acids [178].
Currently, most pilot scale publications evaluate biomass production for a single purpose. This is the case of the pilot plant located in Tarragona (Spain), which consists of six photobioreactors operating in batch mode for cultivation of Nannochloropsis gaditana for the extraction of lipids [179]. In Brazil, the Petrobras research center launched a pilot plant in 2009 to produce biodiesel from microalgae. Currently, it has the capacity to operate open ponds with a total area of 100 m2, producing 6,000L of algae culture [180]. The biodiesel factory Grand Valle Bio Energy Ltda., Located in the state of Rio de Janeiro, made some investments in technological development for the cultivation and use of microalgae as an alternative raw material in the production of biodiesel. This company developed the cultivation of the microalga Monoraphidium sp. in pilot-scale photobioreactors [181].
Sevrin (1998) carried out experiments in a pilot plant for simultaneous production of microalgae, zooplankton and fish. The main objective of the project was to develop a biological treatment in situ for pig manure, which would imply a minimum treatment by the pig farmer, as well as the generation of microalgal biomass. For this, an experimental system of lagoons was established consisting of two algae ponds, two zooplankton ponds and a fish culture pond working in series and in closed circuit. Pollutants like ammonia and the phosphates contained in pig manure are used by the Chlorococcales microalgae, which in turn are consumed by the zooplankton Daphnia magna, the latter being food for the fish. The operation of this system was analyzed during one year, finding better removals of nitrogen and phosphorus [182]. A promising biorefinery strategy was developed in Almeria (Spain), where an agricultural biofertilizer "Algafert" was created through the cultivation of microalgae in wastewater. The microalga biomass obtained is subjected to enzymatic hydrolysis to release the content of essential amino acids, phytohormones and peptides that can be used more quickly by plants (personal communication from scientists at Almeria).
6. Conclusions
The transformation of light into chemical energy and stable biomolecules through photosynthesis carried out by microalgae is one of the processes with the greatest potential to contribute in solving the global challenges facing humanity today. Although these microorganisms offer a broad portfolio of compounds with diverse applications, only a few of them have transcended the frontiers of research towards commercial production and exploitation. The greatest scientific interest derived from recent academic work with microalgae lies in its exploitation as a source of bioenergy, however, multidisciplinary efforts are still required to make this process sustainable and the application of the biorefinery model provides a plausible outlet. Furthermore, it is necessary to join efforts for the development and innovation in these platforms that allow reductions in production costs, but also the incursion in new markets and the development of new products from the same input.