1. Introduction
Cyanide is used in the gold and silver mining industry for the extraction from ore present in the rock and has a great environmental impact -which is hazardous to aquatic life- due to the lack of treatment [1]. These effluents tend to have high concentrations of this poison able to kill many eukaryotes as has an inhibitory effect on respiration due to cyanide binds to the cytochrome c oxidase and many other enzymes triggering a decrease in ATP/ADP ratio [2] and it forms a stable complex with transition metals, disrupting protein function [3, 4]. Thus, the removal of cyanide from wastewater and the environment is desired. In spite of its toxicity, a wide range in the various life forms including some microorganisms can utilize cyanide as substrate [5].
Microbial degradation is an attractive technology for cyanide, turning it into a valuable natural tool for environmental purposes [6] and take advantage of the indigenous microorganisms such as bacteria and their ability to use this chemical as a source of nutrients to metabolize it in safer products like CO2, formate, formamide, and methane [7,8]. The diversity and distribution of microorganisms is influenced by the composition of the geochemical matrix of microhabitats and the introduction of toxic or polluting substances that cause changes in the structure, diversity and function of the microbial community associated with the site [9].
To date, several reports have described the ability of microorganisms to grow on cyanide compounds; bacterial communities such as Pseudomonas, capable of degrading cyanide 10 mg L-1[10] and two isolates of this genus, immobilized on activated charcoal, degrading concentrations up to 340 mg L-1[10,11].Arthrobacter sp., Zoogloearamigera, Acidovorax sp., Achromobacter sp., Janthinobacterium sp., Klebsiella sp., Bacillus pumillus, Burkhoderia cepacia, Alcaligenes sp., Serratia marcescen and Rhodococcus sp., among others, have been reported as cyanide degrading organisms [6, 12-19]. Besides bacteria, species of the archaeal genus Methanosarcina (under anaerobic conditions), some fungi like Fusarium solani and Trichoderma polysporum at pH 4 [20] and some algae like Scenedesmus obliquus[21] have been reported to degrade cyanide.
Microbial ecology in hostile environments is of great interest because it has elucidated information on the fundamental role of microorganisms living there. The ability to resist to high concentrations of this pollutant allows selecting some members that probably have the ability to survive these conditions.
Our aim was to use both conventional and molecular methods as complementary approaches to estimate bacterial diversity present in residual effluents from cyanidation processes in two gold processing plants and evaluate the cyanide degradation potential of four isolated bacterial strains. The molecular tools included temporal temperature gradient electrophoresis (TTGE), and ribosomal intergenic spacer analysis (RISA or IGS) [22-24]. This approach enables the detection of dominant bacterial communities that could be of importance in the treatment of mining wastewater and may offer an alternative method for removing cyanide before it has a chance to contaminate the environment.
2. Materials and methods
2.1 Description of sampling sites and sample processing
Ten samples were taken at two different sites. Samples M1, M2, M3 and M4 were taken from an industrial plant and A5, A6, A7, A8, A9 and A10 from an artisanal plant. Liquid samples of approximately 500 mL were taken, which were stored in sterile, dark and screw-on glass bottles, at a temperature of 4 ° C, for a maximum time of 4 h before being worked.
2.2 Physical-chemical analysis
The pH was measured using a HACH-H130d Multi-parameter probe with an Ag/AgCl electrode. The free cyanide concentration was measured by titration, using an automatic burette and analytical grade 0.005 mol L-1 silver nitrate (AgNO3) as the titrant (0.84935 g of AgNO3 in 1 L of sterile water). 5-(4-Dimethylaminobenzylidene)-rhodanine (Rh-) was used as the indicator, prepared in a solution of 20 mg per 100 mL of acetone, and used according to the “standard methods for the examination of water and wastewater” [25]. The effluent was characterized by atomic absorption spectroscopy [26] in order to determine the individual chemical elements. The total cyanide was measured by using an acid distillation process to eliminate complex cyanides [27] present in the samples. Finally, a titration was performed as described previously.
2.3 Analysis of the bacterial community by TTGE
Bacterial DNA from each sample was extracted using the FastDNA™ SPIN kit for soil [28]. For each sample, two separate extractions were performed and eluates from the same sample were mixed together and then concentrated using a Concentrator 2000 (Eppendorf, USA). DNA was quantified by spectrophotometry using the NanoDrop 2000 version 1.0 (Thermo Scientific, USA).
Three samples from the industrial plant (M2, M3 and M4) and five samples from the artisanal plant (A5, A6, A7, A9 and A10) were processed for TTGE analysis [22,24,29]. The V3-V6 region of the 16S rDNA was amplified with primers 341F with a GC tail at the 5' end, and 907R [30]. The 16S rDNA fragments obtained from the amplification were separated using a DCode Universal Mutation Detection System (BioRad, USA), with a 7% polyacrylamide gel containing 7M urea, in 1.5X TAE buffer. Electrophoresis was carried out at a constant voltage of 55 V for 15 h with an initial and final temperature of 66 and 69°C, respectively, and at a temperature ramp rate of 0.1°C h-1. The gels were stained with SafeView™ (Applied Biological Materials Inc). For the statistical analysis of the TTGE banding patterns, the presence-absence matrix was used to evaluate the richness (S) index and a non-metric multidimensional scaling (NMDS) analysis based on the Bray-Curtis similarity index was performed to observe significant differences between sampling sites. This was done using the PAST Software version 3.0 [31].
Banding patterns were analyzed with the Dice similarity coefficient and unweighted pair group method with arithmetic mean (UPGMA) clustering method, using the GelCompar II software (Applied Maths Biosystems, Belgium). DNA bands with unique migration patterns according to the molecular marker and/or bands with the highest intensity on each TTGE gel, were excised. DNA from each band was recovered by the elution method, leaving the samples overnight in 60 μL of PCR grade water. The eluted DNA was used to carry out the re-amplification, using primers 907R and 341F (without the GC tail). Re-amplified products were verified by visualization in 1% agarose gels and purified using Wizard PCR Preps.
The double-stranded DNA was sequenced in both directions using the ABI PRISM 3700 DNA analyzer service (Applied Biosystems). The sequences were edited using the BioEdit software and compared to reference sequences from the GenBank database, using the BLAST alignment search tool [32], Ribosomal Database Project (RDP) and EzBioCloud 16S database (help.ezbiocloud.net) [33]. Sequences were aligned with MEGA software, version 6 (http: //megasoftware.net/) [34]. Chimeras were analyzed with DECIPHER [35]. All sequences were deposited in the NCBI database under accession numbers KU175879-KU175885.
2.4 Microbiological analysis
Cyanide-degrading bacteria were isolated and purified in LB medium (5.0 g NaCl, 5.0 g yeast extract, 10.0 g casein peptone, 18 g agar, in one liter of distilled water and final pH 7.2 ± 0.2) and in LB medium supplemented with effluent from the industrial plant, containing approximately 1100 ppm of cyanide. For supplemented medium, was used the same composition in a liter of cyanide effluent, with a final pH of 9.5 (± 0.2). This step excluded those bacteria incapable of growing under these conditions. The LB medium was autoclaved at 121°C, for 20 min, and the cyanide effluent was sterilized using a 0.22 μm Millipore filter.
Samples were serially diluted to 1:1000 in sterile distilled water, and plated into Petri dishes with culture medium. All experiments were carried out in duplicate and with a negative control (without inoculum). The Petri dishes were incubated inverted under aerobic conditions, at 30°C, for 24 to 48 h, until colonies were observed. The isolates were named according to the place of origin (A or M) followed by the medium used to obtain it (LB) and finally by the isolate number.
After the colonies were purified by the quadrant streaking method, they were characterized microscopically by gram staining and molecularly by RISA (or IGS) [22-24,29]. RISA banding patterns were resolved by polyacrylamide gel electrophoresis (PAGE) and analyzed with GelCompar II software (Applied Maths Biosystems, Belgium). RISA-PAGE was performed in a Mini-PROTEAN Tetra cell electrophoresis unit, with 7% polyacrylamide gels (acrylamide/bis-acrylamide 29:1), for 100 min, at 130 V. For long-term preservation, all isolates were stored in LB medium, with 20% glycerol, at −20 °C and−80 °C.
2.5 Characterization of cyanide-degrading bacteria by 16S rRNA gene sequencing
The 16S rRNA gene sequence was used to determine the identity of the isolates obtained. PCR products were verified by visualization in 1% agarose gels and purified using Wizard PCR Preps (Promega, Medellin, Colombia). The double-stranded DNA was sequenced in both directions with primers Eubac27F and 1492R [36], using the ABI PRISM 3700 DNA analyzer service (Applied Biosystems, Carlsbad). Sequences were edited using the BioEdit® program [37] and compared to the reference sequences from the GenBank database, using the BLAST alignment search tool [32], the Ribosomal Database Project (RDP) and EzBioCloud 16S database (help.ezbiocloud.net) [33]. Sequences were aligned with MEGA software, version 6 (http://megasoftware.net/) [34]. Chimeras were analyzed with DECIPHER [35] and the phylogenetic tree was generated by the Neighbor-Joining method [38]. Evolutionary distances were calculated using the Jukes-Cantor method and bootstrap of 1000 replicates. All sequences were deposited in the NCBI database under accession numbers KU175866-KU175878.
2.6 Cyanide-degrading activity assays
Four isolates were selected according to the initial cyanide concentrations measured at the sampling sites (high 303.85 ppm, intermediate 147.02 ppm and low 39.02 ppm, Table 1). Isolates M1LQA1, A7R2A9, A7LQA15 and A9LSA19 were reactivated in LB medium, for six days, to a cell concentration greater than 109 cells mL-1, at 30°C (the average temperature of the sampling site) and 180 rpm on an orbital shaker. Under same conditions, the commercial strain Pseudomonas fluorescens DSM 7155 (Leibniz Institute DSMZ-German Collection of Microorganisms and Cell Cultures) was activated and used as a positive control in bioassays.
Table 1. Measurement of the initial physical-chemical parameters (pH and cyanide concentration) of the samples (A), artisanal plant and (M), industrial plant.
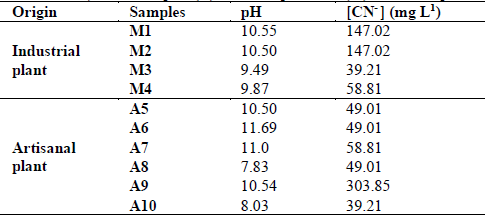
Source: The authors
In 500 mL Erlenmeyer flasks, 250 mL of LB medium were inoculated and supplemented with 200 ppm NaCN, and adjusted to pH 10. For the positive control and four selected isolates, 10 mL of live inoculum was added to 240 mL of LB medium, supplemented with 200 ppm NaCN, for a final volume of 250 mL. Flasks with 250 mL of LB medium supplemented with NaCN, without inoculum, were included as negative controls. The cultures were grown in duplicate in an orbital shaker (180 rpm, 30°C), for 72h, under aerobic conditions. A 10 mL aliquot was extracted every 24 h to measure pH and cyanide concentration. Biomass was measured at the end of the test [39].
The biologically degraded cyanide was determinated through mass balance with equations 1 and 2 [40].
Where:
CN- B: Biologically degraded cyanide (mg CN- L-1)
CN- S: Inicial free cyanide concentration (mg CN- L-1)
CN- R: Residual free cyanide in the media (mg CN- L-1)
CN- V: Cyanide volatilised (mg CN- L-1)
Where:
CN- Vo: Inicial cyanide concentration in the control cultures (mg CN- L-1)
CN- Vf: Final cyanide concentrations in the control cultures (mg CN- L-1)
The experimental error was estimated and represented with error bars (media) of the test average. Data were analyzed using SAS® STUDIO software, version 3.4 (Basic Edition). An ANOVA test was performed (95% confidence interval) verifying F> Pr.
3. Results
3.1 Sample analysis
Cyanide concentrations ranged from 39.21 to 147.02 ppm, in samples taken from the industrial plant, and between 39.21 and 303.85 ppm in the artisanal plant. The pH was alkaline in almost all samples, with the exception of sample A8 (Table 1). Table 2 shows the results of the chemical analysis of the effluent used to supplement the culture media for isolation of microorganisms. This revealed the presence of, in addition to fair amounts of cyanide, the presence of important proportions of Ca and Zn, minors of Cu and iron, and traces of Pb and Al. (Table 2). These conditions of cyanide and heavy metal concentration, where the samples were taken, show that the isolates were cyanide tolerant.
3.2 Determination of bacterial diversity through TTGE
The evaluation of the diversity of bacterial communities associated with industrial and artisanal plants was determined assuming that each band with a different migration pattern represented a unique bacterial group (Fig. 1). The evaluation of the migration profiles, revealed a low diversity, with few bands and presence of several migration patterns.
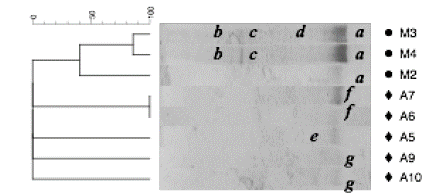
Source: The authors
Figure 1 Dendogram and TTGE gel banding patterns of 16S rDNA PCR products from the total fraction of samples taken from industrial (M) and artisanal (A) plants. Positions (a, b, c, d, e, f, g) in the lanes represent the bands selected for identification. The identity of each band is shown in the Fig. 2.
The seven bands were selected for re-amplification, and a sequence of approximately 500 bp was obtained for each band, showing differences in both their migration profiles and molecular identifications. Specifically, band a, was identified as a non-cultivable member of the Comamonadaceae family, and was present in three out of the four industrial samples (M2, M3, and M4), suggesting that the bacterial community in the industrial plant is dominated by that specific phylotype. Band f was identified as member of the Bacillus, and it was observed in common for samples A6 and A7, corresponding to thickening and sedimentation tanks, respectively. On the other hand, band g, identified as a member of Pseudoalteromonas, was shared by samples A9 and A10. These samples corresponded to sites where cyanide solutions were added to an effluent. The bands b, c, d, e were identified as Erysipelotrichaceae, member of phyla Firmicutes, Hydrogenophaga and Halomonas, respectively (Fig. 2).
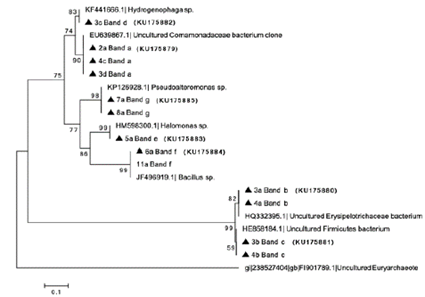
Source: The authors
Figure 2 Phylogenetic distance tree based on 16S rDNA gene V3-V6 sequences (TTGE) from the artisanal and industrial gold processing plants with the accession number. The dendrogram was generated with the DICE similarity index and unweighted pair group method with arithmetic mean (UPGMA) clustering method.
Sample A9 was near where the cyanide was added to the effluent and sample A10 was 1.5 m downstream of it. There were also individual bands in the TTGE that could not be characterized because of their low intensity.
Profiles were evaluated using the Dice similarity coefficient, which revealed differences between the two gold cyanide leaching plants. Since, the samples formed two clusters, one for each plant. Further, an analysis of similarity (ANOSIM) revealed that there are significant differences (R = 0.2313) between the processing plants, according to Clarke (1993) [41], who demonstrated that positive values of R up to 1 indicate dissimilarity between groups. Additionally, the NMDS analysis of the TTGE displayed differences between the two sampling sites, since these grouped according to their origin (Fig. 3).
3.3 Identification of isolates
The isolates were characterized microscopically by gram staining and molecularly by RISA (or IGS). All strains, excluding the A9LBA21, were gram positive. RISA analyses showed differences in the pattern of bands. Twenty isolates were identified by 16S rDNA sequencing Fig. 4. Culture dependent methods displayed genera, such as Bacillus sp., Rhodococcus sp., Microbacterium sp., Alcaligenes sp., Lysinibacillus sp., Pisciglobus sp., Trichococcus sp., Paenibacillus sp., Enterococcus sp., Aerocuccus sp., Brachybacterium sp. and Tessaracoccus sp.
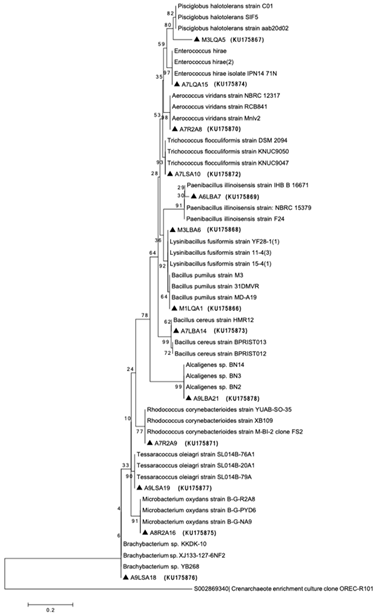
Source: The authors.
Figure 4 Phylogenetic location of bacterial isolates based on 16S rRNA gene sequences from the artisanal and industrial gold processing plants with the accession number. The dendrogram was generated by the Neighbor -joining method in MEGA, using Kimura -2 with 1000 replicates in the bootstrap analysis. The 16S rRNA gene sequence of Crenarchaeota was used as an “out -group”.
3.4 Assay for cyanide-degrading activity by bacterial isolates
The four bacterial isolates were selected, based on the cyanide concentration, showing Rhodococcus sp. (M1LQA1) and Enterococcus sp. (A7R2A9) for the low cyanide concentration (58.81 ppm) effluent, Bacillus sp. (A7LQA15) in the intermediate cyanide concentration effluent (147.02 ppm), and Tessaracoccus sp. (A9LSA19) found in the highest cyanide concentration (303.85 ppm) effluent (Table 1). In addition to native strain isolates, a commercial strain of Pseudomonas fluorescens (DSM 7155) was used as positive control in cyanide degradation assays.
In this work only native strains were used along with one Pseudomonas reference strain. This strain was chosen because other authors have shown that it degrades cyanide due to the enzyme nitrilase capable of degrading cyanide, and thus, is widely used for this purpose [4,10,11,16,42,43].
The four isolates showed a degradation of cyanide concentration, evidenced for its reduction in time in the Fig. 5, when compared to the un-inoculated controls. The growth kinetics of the isolates revealed that a significant degradation occurred within the first 24 h, without an evident lag or adaptation phase. The essays that showed the highest cyanide degradation were those using isolates with Bacillus sp., capable to degrade 41.9 mg CN- L-1, and Enterococcus sp. capable to degrade 27.5 mg CN- L-1. According to the mass balance, the percentages of removal efficiency were of 21% and 14%, respectively. Similar results were observed in the essays using the strain P. fluorescens (DSM7155).
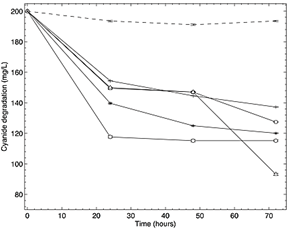
Source: The authors
Figure 5 Degradation plot of cyanide concentration vs time in hours in LB medium using Bacillus pumilus (Bp), Rhodococcuscorynebacterioides (
Rc), Enterococcus hirae (
Eh) and Tessaracoccusoleagri (
To) isolates, a positive control with the strain P. fluorescens (DSM7155) (
PfC +) and a negative control without inoculum (
Control).
On the other hand, isolates of Rhodococuss sp., Tessaracoccus sp. and P. fluorescens, showed removal efficiencies of 11%, 12.4% and 16%, degrading 22, 25 y 32 mg CN-l-1, respectively, according to the mass balance. The assay had a final pH of 9.45 for the isolates Bacillus sp and Rhodococuss sp., 9.43 for Enterococcus sp. and 9.49 for Tessaracoccus sp. (Fig.6). Finally, the positive control had the lowest final pH of 9.27. Cyanide volatilization was considered in the mass balance. ANOVA returned values F>Pr, accepting the alternative hypothesis, revealing differences between the average of the treatments. Thus, at least two treatments were different from each other, when degrading cyanide.
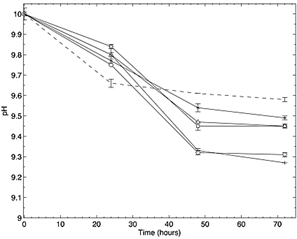
Source: The authors.
Figure 6 Change of pH in the time lapse of the degradation assay in LB medium using Bacillus pumilus (Bp), Rhodococcus corynebacterioides (
Rc), Enterococcus hirae (
Eh) and Tessaracoccus oleagri (
To) isolates, a positive control with the strain P. fluorescens (DSM7155) (
PfC +) and a negative control without inoculum (
Control-).
4. Discussion
In this research, the bacterial diversity associated with two gold mining industries in Colombia was examined using both culture-dependent and culture-independent techniques, with variations in the bacterial populations were identified based. The bacterial communities in all samples were dominated by two specific phylotypes: Proteobacteria and Firmicutes. However, by culture-dependent analysis, members of the phylum Actinobacteria were also detected, suggesting low abundance of this group. TTGE- culture-independent analysis showed differences in diversity among sampling mines (Fig. 1). This was expected, since each plant (industrial plant and the artisanal plant) presents different types of physical and chemical variables that likely select for different types of bacteria [44]. The industrial effluent was characterized and showed the presence of zinc, lead, iron, copper, aluminium and calcium (Table 2). Calcium was the metal with the highest concentration in the effluent, probably due to the lime (Ca(OH)2) commonly used to keep a high pH in the cyanidation tank [4]. Zinc comes from sphalerite, which is found at low concentrations in the mineral and could come from its partial leaching, which is common in lower concentrations in the Marmato District’s mineral. Another source of Zn is the metal zinc used in the cyanidation tank to precipitate the gold after leaching. The iron detected was probably due to the partial leaching of the sphalerite (present in Marmato as marmatite, a variety rich in iron) and chalcopyrite, as well as other minerals present in the deposit such as pyrite, pyrrhotite, arsenopyrite, among others. Their low concentration could be due to: (i) the low solubility of pyrite (in high percentage in the mineral) in the aqueous solution, (ii) the low concentrations of iron in the sphalerite, the partial dissolution of chalcopyrite and the minimum proportions of pyrrhotite and arsenopyrite in the mineral. Lead and copper could come from the partial leaching of galena and chalcopyrite minerals, respectively, present at the Marmato deposit. Finally, aluminium could come from the partial leaching of some aluminosilicates present as plagioclase, among others. These conditions of cyanide concentration and metals composition assuring the isolates were at least cyanide tolerant.
Differences in the cyanidation processes seem to influence the predominant populations present in each sample, depending on the place of origin. This was confirmed by NMDS analysis (Fig. 3), which determined the characteristics of the banding patterns from both sampling sites; in addition, it was observed that the characteristics of the samples were more homogeneous in samples from the industrial plant, due to the regulation of these variables in this plant. In general, there was greater heterogeneity among the samples of the artisanal plant than those of the industrial plant, probably due to the little control in the physical and chemical parameters of the cyanide leaching process, such as pH, temperature, and cyanide concentration.
The genera Bacillus, Rhodococcus, Microbacterium and Alcaligenes have been reported in cyanide-contaminated sites and found that their metabolism was associated with cyanide degradation [13,19,44-47]. However, other isolates that have not yet been associated with cyanide metabolism, but have been found in some harsh conditions, have been identified, such as Lysinibacillus sp. (KU175868), Pisciglobus sp. (KU175867), Trichococcus sp. (KU175872), Paenibacillus sp. (KU175869), Enterococcus sp. (KU175874), Aerocuccus sp. (KU175870), Brachybacterium sp. (KU175876) and Tessaracoccus sp. (KU175877). Although they have not yet been reported as cyanide-degrading microorganisms, since they are microorganisms found in different extreme environments, and are versatile and adaptable, it is possible that their metabolism has the ability to assimilate cyanide as a source of carbon and/or nitrogen.
It was found that Bacillus sp. was the isolate with the best degradation capacity, with 21% of efficiency of cyanide degraded, according to de mass balance. This isolate was found in a similar concentration of cyanide in the effluent and it could adapt easily to the conditions. Several members of the genus Bacillus sp. and especially the B. pumilus species, have been reported as good cyanide degraders [48-50] and have been widely used for these types of experiments. The strain Enterococcus sp., which showed good growth in the medium and similar degradation (14%) that the positive control (P. fluorescens), belongs to the family Enterococcaceae. Previous studies suggest that other bacterial strains from this family are capable of growing under anaerobic conditions and in the presence of complex cyanide compounds, being that this capability is attributed to the proton-motive force to perform reductive processes [51].
Tessaracoccus sp. isolate, with an intermediate efficiency of degradation of 12.4%, has not been reported as cyanide resistant or as a cyanide degrader. However, some members of the family degrade toxic compounds. For example, the Propioniferax sp., degrades phenol [52]. Although the cyanide degradation pathways for Enterococcus sp. and Tessaracoccus sp. are not known yet, they may owe their degradation capacity to their ability to adapt and survive in places where cyanide and heavy metals are present, a common adaptation feature in Gram positive bacteria [53]. On the other hand, for Rhodococcus sp. (with an efficiency of 11%), studies reported that other members of this strain were able to degrade up to 50% cyanide in culture media with glucose and nitrogen [46, 47, 54].
Assays with Enterococcus sp., were the first to arrive at its stationary phase of degradation, reaching its greater reduction of cyanide concentration at 24 h. A similar behavior was observed in the assays with Tessaracoccus sp., where the highest rate of degradation occurred in the first 24 h, and degradation in the following hours was minimal, which could suggest that cyanide degradation was initiated by constitutive rather than inducible enzymes. On the contrary, trials with isolate Rhodococcus sp. experienced two degradation phases, one during the first 24 h and another after 48 h, with an intermediate phase (between 24 and 48 h) where there was no degradation. This could be explained by it possesses a probable path for cyanide degradation through the hydrolytic route utilizing the enzymes nitrile hydratase and amidase, where both are responsible for cyanide metabolism. [55]. Usually, the cyanide degradation is carried out in short time periods, that is, in hours and even in minutes [49]. In that time, some species can develop stages according to their metabolism.
The degradation assay indicated that the best cyanide degrading isolates were Bacillus sp. and Enterococcus sp., reaching 21% and 14% of efficiency in cyanide removal percentages, respectively. However, it is necessary to evaluate other conditions of the culture media such as carbon source, and nitrogen source, which may favor bacterial growth and increase cyanide degradation. Thus, we conducted a trial using LB medium, because some reference works demonstrate that this sort of medium biostimulates the microbial growth and improves the performance when compared to medium without carbon and nitrogen sources [56-59]. The four isolates were assessed in a mineral medium (M9) and the results showed they can assimilate cyanide, but performance was low compared to LB medium (data not shown).
5. Conclusion
In this study, the cyanide degrading bacteria, that were found in cyanide gold leaching plants in Colombia, offer a promising alternative to remove this toxicant by biological degradation, before it has a chance to enter the environment. Some of the potential benefits of the biological degradation process, as an alternative to conventional methods used on an industrial scale, are: i) no addition of toxic or costly chemicals, and ii) no additional requirement for pH controls, since microorganisms work in the conditions in which an industrial plant operates.