1. Introduction
Cellulose gum (CG), xanthan gum (XG) and guar gum (GG) are hydrophilic polysaccharides, also called hydrocolloids, used in various industries as stabilizers and rheology modifiers with appealing properties for different uses [1]. Hydrocolloids used in food applications exhibit a viscoelastic rheological behavior. This behavior arises from the chemical structures of hydrocolloids, which form dispersions in aqueous mediums, allowing them to resist strain as evidenced in solids. However, when the stress applied exceeds the elastic resistance threshold, the material transitions into a flowing state, exhibiting characteristics of a viscous liquid [2]. During this flow phase, hydrocolloid dispersions conform to non-Newtonian shear-thinning fluid behavior. When hydrocolloids are combined to form a solution in aqueous media, the gum structures interact with each other, leading to alterations in viscoelastic behavior (formation of gels or high-viscosity solutions). Importantly, the changes in shearing often do not follow a proportional pattern in the mixture, but instead lead to an amplification of specific effects. This phenomenon is commonly referred to as a synergistic effect [3].
CG, also known as sodium carboxymethyl cellulose, is a polysaccharide consisting of cellulosic structures linked by an ether group to an ionic carboxylic radical, which is produced by the reaction of cellulose with sodium hydroxide (generating the basic form of cellulose) and subsequently reacting with chloroacetic acid [4]. CG is derived from cellulose and is widely used due to its solubility in water, which imparts it with the characteristic properties of being a hydrocolloid, making it valuable as a thickening agent, viscosifier, suspension agent, stabilizer, and film former. It can be used in detergents, food, textiles, paints and inks, glues, pharmaceuticals, cosmetics, ceramics, paper, and in the oil industry, among others [5]. For its use in the food industry, manufactured CG has a minimum degree of purity sodium glycolate. Cellulose gum is used in dressings and sauces, honeys and syrups, ice cream and creams, diet foods, ready-to-drink beverages, powdered drinks, and bakery and confectionery products, among others. XG is an extracellular hydrophilic anionic polysaccharide of high molecular weight produced by several species of Xanthomonas, with Xanthomonas Xampestris B-1459 as the bacterium commercially used for its synthesis [6,7]. The backbone of the polysaccharide chain is composed of β-D-glucose units linked through the first and fourth positions. In the third position of the main chain, trisaccharide chains consisting of two mannose groups and a glucuronic acid are alternately linked. Around half of the terminal mannose units have a pyruvic acid group attached to their fourth and sixth positions. The mannose units attached to the main chain have an acetyl group at the sixth position [3]. XG is a non-gelling biopolymer formed in aqueous media with an ordered rigid chain formation; it is highly soluble both in hot and cold water and can form high viscosity solutions, even at low concentrations [8]. Due to its temperature and pH stability, it is widely accepted in the industry [9]. XG aqueous solutions undergo a conformational transition with increasing temperature, which is associated with a change from a rigid and ordered structure to a more flexible and disordered structure at high temperatures. This conformational change was first noticed by the sigmoidal variation of viscosity with temperature [8]. CG is obtained from the seed endosperm of Cyamopsis tetragonolobus, a plant belonging to the legume family that grows in arid or semi-arid areas of India, Pakistan and specific areas of Texas and Arkansas [10]. GG is a polysaccharide consisting of a straight chain of mannose units linked on the sides with single galactose units at a 2:1 ratio (mannose:galactose) [11]. An important property of GG is its ability to hydrate quickly in cold water and to produce highly viscous solutions. The viscosity GG imparts to a solution depends on various factors including time, temperature, concentration, pH, ionic strength, and the type of agitation [2,3,11].
The synergistic properties arising from hydrocolloid mixtures hold great promise for the development of new products and compounds for various industrial applications, characterized by their ability to exhibit high viscosities and enhanced stability under diverse operating conditions [1,9]. These conditions may encompass saline, acidic, or alkaline environments, with varying concentrations of solids, and a range of usage temperatures, among other factors. For some industrial applications, the CG produced in the Colombian industry lacks the necessary properties to serve as a suitable rheological additive in food applications such as powdered soft drinks, industrially processed fruit drinks, sauces, dairy products, bakery products, and some non-food applications such as coatings or paints used in construction or even drilling fluids for the oil industry. This is primarily attributed to the requirement of attaining precise viscosity levels and specific rheological behavior within specified dosage limits. For instance, in some food applications, such as sauces and dairy products, there are restrictions on the maximum allowable dosage of hydrocolloids [12] and CG may not be the sole product capable of meeting the expected performance. To address this limitation, other hydrocolloids are used, including XG and GG, are employed to contribute to the application of the desired effect sought with rheological additives. In most cases, particularly in the above-mentioned applications, a combination of the gum options gum mixtures are used, rather than relying on a single gum product alone.
An analysis of research related to hydrocolloid synergies, such as natural gums, thickeners, and rheology conformers, reveals studies exploring synergistic interactions among various components, including GG, CG, carob bean gum, alginates, and pectin, under specific conditions [9,13-15]. However, there is little research on Colombian-produced CG mixtures. Thus, from a technical and economic standpoint, the study of hydrocolloid mixtures is of great interest, since it can offer information about the rheological properties of individual products and their applications. This can provide insights into how to reduce costs by using less active material per component, especially with high-cost gums that are difficult to obtain. Similarly, given the numerous applications where gums serve as thickeners and stabilizers in different temperature-dependent media [16], this study is of great utility in examining rheological behavior across various aqueous medium conditions.
2. Materials and Methods
2.1. Materials
The following raw materials were used in this study: cellulose gum (CG) commercial brand Gelycel® of Amtex S.A. produced in Colombia; xanthan gum (XG) produced in China by Deosen Biochemical Ltd.; and guar gum (GG) produced in India by Durga Enterprises.
2.2. Gum characterization
To assess the conformity and initial characteristics of CG, XG, and GG, the hydrocolloids were analyzed for the following physicochemical parameters: moisture content, purity, Brookfield LV viscosity, and pH in solution at 1%.
2.3. Gum preparation
To prepare the samples prior to dissolution, specific amounts of each gum were weighed according to the proportion of the mixture, totaling 50g in weight. Subsequently, 500 g of distilled water was added to each sample and stirred until complete dissolution, ensuring that the temperature did not exceed 25°C. After complete dissolution, stirring is stopped, and the sample is left to settle for one minute. This process yielded three single-component mixtures, twelve binary composition mixtures, and six ternary composition mixtures, as indicated in Table 1.
2.4. Rheological analysis
Regarding gum characterization, viscosity determination was carried out using a Brookfield LV rheometer following the manufacturer's recommended parameters [17] and in accordance with the ASTM D-1439 standard [18].
Anton Paar MCR-301® rheometer with denominated geometry CP-50 1° with 50 mm in diameter and 1° with the following conditions depending on the type of analysis:
Flow curve: Flow curve experiments were conducted within a shear rate range of 0.1 and 100 s -1 in 300 s, and at a shear rate, the shear stress, and the apparent viscosity. Experimental flow curves were fitted using two models: the Cross model given by Eq. (1):
Dynamic rheological tests were performed using an
Table 1 Compositions of the mixtures evaluated (in mass fraction)
Mix number | CG | XG | GG |
---|---|---|---|
|
|
|
|
Source: the authors.
where 𝜂 is the apparent viscosity; η0 is the Newtonian viscosity measured at low shear rates; η∞ is the Newtonian viscosity measured at high shear rates; 𝐾𝐶𝑟 is the Cross model constant (measured in time units); and 𝑚 is a dimensionless constant. The second model is the power law model given by Eq. (2):
where η is the apparent viscosity; τ the shear stress;
the shear rate; K the coefficient of consistency, and n the flow performance index (n-1 < 0 corresponds to a shear-thinning behavior, n-1 > 0 corresponds to a shear-thickening behavior, and n = 1 corresponds to a Newtonian behavior).
Thixotropy curve: Thixotropy curves were generated at a constant temperature of 25°C involving a sequence of shear rate variations: an ascending shear rate slope of 1 to 100 s -1 over 300 s, a constant rate of 100 s -1 for 300 s, and a descending slope from 100 to 1 s -1 over 300 s. Using the rheometer software, graph depicting the relationship between shear stress and shear rate was used to calculate both the area of the upward curve and the area of hysteresis.
Oscillatory tests: The amplitude sweep was conducted at 25°C, covering a strain range (γ) from 0.05 to 1000%, and a constant frequency (f) of 1 Hz. The frequency sweep was conducted at 25°C at a frequency range (f) from 100 to 1 Hz and a constant strain (γ) of 0.5%. The temperature sweep was conducted within a range from 10 to 70°C (3°C/min) and at a constant strain and frequency of 0.5% and 10 Hz, respectively. The rheometer software yielded the elastic modulus (G’), the viscous modulus (G”), the phase angle (δ), and complex viscosity (η*).
The dependence of viscosity and, in general, of the rheological parameters on temperature was determined by modeling using the Arrhenius Eq. (3) [2,19]:
where A is the pre-exponential factor, Ea is the activation energy (J·mol-1), R is the universal gas constant (J·mol -1·K -1), and T is the absolute temperature (°K) [20]. The parameter B can be any rheological parameter of interest to be analyzed as a function of temperature, such as the dynamic viscosity when performing rotational tests [21], complex viscosity or the elastic, viscous, or complex moduli resulting from oscillatory tests.
2.5. Experimental design and statistical analysis
Experimental analysis followed a simplex-lattice mixture design of 3 components (CG, XG, and GG), of order 5 and with central points, resulting in 21 points as shown in Table 1.
Data analysis for the respective mixture designs was carried out using Minitab® software with the Design of Experiments (DOE) tool, within the mixture design section. The computational model selected to analyze the response variables (viscosity, thixotropy, elastic modulus, viscous modulus, phase angle, and complex viscosity) in the different mixture designs employed a full cubic order model (see Eq. (4)). This approach enables the design to be adjusted to a first, second, or third order model allowing the modeling of extreme response changes as the proportion of one or more components approaches its limit [22]:
where E(y) is the expected or adjusted value of the response variable according to experimental data, β corresponds to the adjustment factors of the model, and xi, xj and xk correspond to the compositions or mass fractions of the gums [23]. The type of model selected is adjusted to a 0.95 level of confidence and verified by the correlation coefficient R2 for each case.
Furthermore, non-linear regression analysis was performed using a statistical tool. This involved inputting the model equation, and the software automatically calculated the constants within the equation, generated data balancing graphics, and determined the standard error (S) of the model regression with a 95% confidence level.
3. Results and discussion
3.1. Gum characterization
Table 2 shows the main properties of each gum used in the study. The purity of CG falls within the parameters suitable for use in food according to the US FDA guidelines, which establish a minimum purity of 99.5% [24]. XG and GG also meet the specifications suitable for consumption in food applications according to FAO parameters, which specify a minimum purity for XG of 91% [25] and for GG of at least 80% [26].
Table 2 Physicochemical analysis of gum.
Parameter | Gum | ||
---|---|---|---|
CG | XG | GG | |
Moisture [%] | 6.6 | 10.6 | 10.5 |
Purity or Gum Content [%] | 99.7 | 91.5* | 84.2* |
Brookfield Viscosity LV, 25°C, [mPa·s] | 5060 | 2924 | 6280 |
pH, 1% solution | 7.20 | 6.91 | 5.80 |
* Data obtained from the manufacturer's certificate of analysis.
Source: the authors.
3.2. Rheological analysis
3.2.1. Apparent viscosity
The apparent viscosity behavior (measured with the Brookfield LV at 30 rpm) for the CG - XG - GG system mixtures is illustrated in Figure 1 across 3 stages: immediate (0 h), at 4 hours, and at 24 hours at rest.
Notably, the highest system viscosities (dark green) are found in the binary interactions CG-GG at a 20:80 ratio, reaching viscosity values above 8000 mPa·s without variation over time. The synergistic interaction of high viscosity between CG-GG is maintained up to ratios of 40:60. Beyond this composition with an increased proportion of CG, viscosity decreases.
The Brookfield viscosity model at 30 rpm and 0 hours is presented in Eq. (5) as a function of the gum compositions, and it exhibits a coefficient of determination R2 of 0.971.
3.2.2. Viscosity model
The observed unphysical values may be attributed to the software used for nonlinear regression, potentially leading to biased fits or local minima rather than global minima [27]. As indicated in Table 3, the power law model exhibits lower error variances, making it the most suitable choice for compositions where XG and GG are predominant, and CG is present in smaller proportions. This behavior is probably due to the high pseudoplasticity of the system generated by the synergies between XG and GG. In such mixtures, the flow performance index n in the power law model tends be below 0.40, indicating a rapid decrease in viscosity with minor changes in shear rate. This evinces high pseudoplasticity, indicating the absence of a Newtonian region at low shear rates and rendering the Cross model unsuitable for said compositions. Numerically, it is apparent that the viscosity value at near-zero shear rates (η0) in the Cross model tends to be high and while viscosity to high shear rates (η∞) tends to small or even negative. Fig. 2 illustrates the data points analyzed within the experimental design and showcases the viscosity model that best aligns with the different compositions.
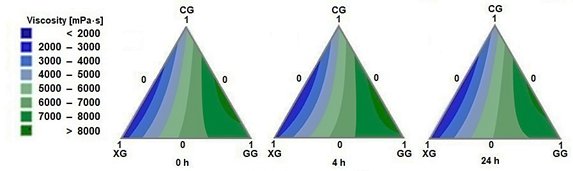
Source: the authors.
Figure 1 Contour plots for viscosity, CG: cellulose gum, XG: xanthan gum and GG: guar gum.
Table 3 Parameters of the mixtures according to the Cross Model and Power Law Model.
Mixture No. | Cross | Power law | |||||||
---|---|---|---|---|---|---|---|---|---|
Ƞ 0 [Pa·s] | Ƞ ∞ [Pa·s] | K cr [Pa·sm] | m [Adim] | Standard error (S) [Pa·s] | k [Pa·sn] | n [Adim] | Standard error (S) [Pa·s] | ||
1 | * | * | * | 0.000 | - | 14.04 | 0.181 | 0.2947 | |
2 | 49.53 | 0.045 | 1.96 | 0.807 | 0.0982 | 15.25 | 0.556 | 1.8972 | |
3 | 19.43 | 0.063 | 1.20 | 0.689 | 0.0589 | 7.86 | 0.652 | 0.8829 | |
4 | 108.91 | 0.005 | 5.73 | 0.792 | 0.1828 | 15.99 | 0.426 | 1.3437 | |
5 | * | * | * | 0.154 | - | 20.92 | 0.224 | 0.4082 | |
6 | 77.88 | 0.192 | 2.43 | 0.803 | 0.1590 | 21.25 | 0.533 | 2.4244 | |
7 | 13.24 | * | 1.91 | 0.603 | - | 4.17 | 0.630 | 0.3611 | |
8 | * | * | * | 0.755 | - | 32.37 | 0.373 | 2.7273 | |
9 | * | 18.05 | 0.784 | -0.037 | - | 9.26 | 0.257 | 0.2952 | |
10 | 86.79 | 0.543 | 2.47 | 0.881 | 0.3169 | 23.83 | 0.515 | 2.9783 | |
11 | 14.97 | 0.024 | 1.37 | 0.654 | 0.0452 | 5.68 | 0.648 | 0.5828 | |
12 | 53.90 | 0.168 | 2.12 | 0.772 | 0.1299 | 16.00 | 0.561 | 1.7908 | |
13 | * | * | * | 0.218 | - | 26.11 | 0.325 | 1.8033 | |
14 | * | * | * | 0.159 | - | 20.72 | 0.210 | 0.5925 | |
15 | * | * | * | 0.194 | - | 28.48 | 0.381 | 1.9545 | |
16 | * | * | * | 0.107 | - | 11.29 | 0.351 | 1.0578 | |
17 | 30.46 | * | 7.27 | 0.545 | - | 3.53 | 0.539 | 0.1506 | |
18 | 34.10 | 0.119 | 1.71 | 0.735 | 0.0794 | 11.43 | 0.598 | 1.2685 | |
19 | 37.93 | 0.478 | 2.05 | 0.885 | 0.1668 | 11.78 | 0.545 | 1.4771 | |
20 | 92.36 | 0.550 | 3.16 | 0.943 | 0.2566 | 21.93 | 0.465 | 2.8086 | |
21 | 38.93 | 0.326 | 3.13 | 0.847 | 0.1775 | 9.28 | 0.499 | 0.9826 |
* Values unphysical.
Source: the authors.
3.2.3. Thixotropic behavior
The thixotropy percentage in the ternary system CG - XG - GG was calculated by measuring the hysteresis between the ascent curve and the descent curve for each prepared mixture. The adjustment model was created with the thixotropy data within the ternary composition diagram (Fig. 3). It is evident that GG is the component that primarily influences thixotropic behavior. As the ternary system contains a higher proportion of a specific gum, the synergy in terms of thixotropy percentage is amplified. Thixotropic behavior can also be observed in binary mixtures of CG and CG, which present thixotropies between 5 and 6%.
The equation that links thixotropy (T [%]) as a function of the gum compositions with a correlation coefficient R2 of 0.931 is shown in Eq. (6).
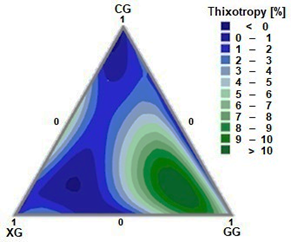
Source: the authors.
Figure 3 Thixotropy of the ternary system; CG: cellulose gum, XG: xanthan gum and GG: guar gum.
3.2.4. Viscoelastic behavior
The viscoelastic behavior, as determined through oscillatory rheology, reveals two important phenomena that merit identification: a) At very low strain levels, it becomes possible to discern the type of structure within the ternary system CG - XG - GG. This helps elucidate the behavior of the internal structure comprising the gum system in aqueous solutions. b) Oscillatory measurements help to assess the stability of the formation of the internal structure over time. In the former case, the internal structure exhibits either elastic solid or viscous fluid behavior, which can be observed through oscillatory amplitude tests. In the latter case, where the stability of viscoelastic structures over time is assessed in mixtures, the characteristics of the internal structure are determined through frequency tests [28,29].3.2.4.1 Oscillatory Amplitude Tests
Amplitude tests enable the characterization of the elastic modulus (G’) and the viscous modulus (G”) of the ternary system. Row a) in Fig. 4 illustrates the behavior of G’ at a strain of 10% (located within the viscoelastic linear region), 50% (elastic solid to viscous fluid transition region) and 100% (strain at which most mixtures are in the melt flow region). High values of G’ with a greater presence of GG, medium values of G’ with a greater presence of XG, and low values of G’ with a greater presence of CG are all obtained at low strain values. As the strain increases (50 and 100%), the values of G’ decrease. At a high strain, the largest G’ is obtained with the binary mixture GG-CG. Row b) in Fig. 4 shows the behavior of G” for strains at 10, 50, and 100%. The gum mixtures that evince high values of G” are found in binary mixtures of GG-CG, and low values of G” are found in binary mixtures of XG-CG. Values of G” remain unaffected by the increase in strain.
Furthermore, the relationship between G’ and G” can be established through the phase angle defined as tan δ = G”/G’. Fig. 5 shows the phase angle (δ) of the viscoelastic linear region (10% strain) for the fabricated mixtures. The areas with the lowest phase angles correspond to those areas where behavioral dominance is more rigid (blue regions); this is mainly due to the presence of XG. Whereas the region with phase angles of around 45° (dark green areas) correspond to the mixture systems where behavior tends to be more fluid; that is, with a predominance of CG in the mixture. Generally, the ternary mixtures of these gums behave as viscous fluids (G” > G’).
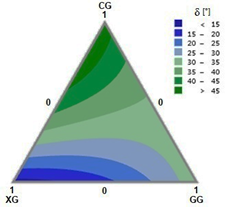
Source: the authors.
Figure 5 Plot of phase angle at the viscoelastic limit (VEL) of CG-XG-GG ternary mixtures at 10% strain.
The phase angle adjustment model (δ) in relation to the composition of the ternary system is shown in Eq. (7) with a correlation coefficient R2 of 0.958.
3.2.4.1 Oscillatory Frequency Tests
Frequency tests are used to determine the stability of the suspension with respect to its structural conformation in certain time periods. High frequencies will indicate stability in short time periods, whereas low frequencies will correspond to longer time periods. Fig. 6 a) shows the values of G’ in the ternary diagram CG-XG-GG for a frequency sweep of 10, 1, and 0.1 Hz. As we can see, G’ is modified in different magnitudes depending on the composition of the gum mixtures. In general, G’ diminishes as the frequency also decreases. This indicates that, in large time intervals, the system exposed to constant strain tends to flow. Nevertheless, the mixture intervals that showed higher values of G’ in the amplitude tests are the ones that now show the greatest variations in G’ as frequency decreases.
In contrast, binary mixtures of XG and CG with lower values of G’ at high frequencies resist variations as the frequency decreases. This is evident, for example, in mixtures with a high GG content, where G’ is high, but where there is an accelerated decrease as frequency diminishes, indicating that material rigidity is unstable over long time periods. Mixtures with a high XG content have low G’, but a decrease in frequency has a small impact on them, which is why these systems are more stable over time.
Fig. 6 b) shows the gum mixtures that are affected to a greater or lesser extent over time with respect to viscoelastic behavior; that is, the phase angle (δ). In general, the presence of XG affects the suspension by providing a more solid structure over a long time period (high frequencies).
3.2.4.2. Oscillatory Temperature Tests
The effect of temperature on the behavior of the ternary system CG-XG-GG can be described by the evolution of G’ depending on the temperature (Fig. 7). The regions with lower G’ values comprise regions of the binary mixture CG-XG, while the regions with higher G’ values correspond to the mixture CG-GG. It is also noted that the gum mixtures with higher G’ values are more sensitive to an increase in temperature. Although the G’ value describes the rigidity of the material, a complex viscosity analysis can also appropriately describe the relationship between the elastic and viscous component. Complex viscosity is a term describing the response to oscillatory sheer stress and associates the elastic and viscous components of the structure in terms of dynamic viscosity.
By analyzing the system using complex viscosity and applying the Arrhenius equation, we obtain the activation energies for each mixture point. Fig. 8 shows the activation energy values for each mixture point. Mixtures with a high XG content have regions with low activation energies (dark blue); and mixtures with high CG and GG contents have regions with high activation energies (dark green). Low activation energies are associated with a lower sensitivity of the analyzed rheological property (in this case, complex viscosity) with respect to temperature [20]. On the other hand, high activation energies indicate more rapid changes in viscosity [30].
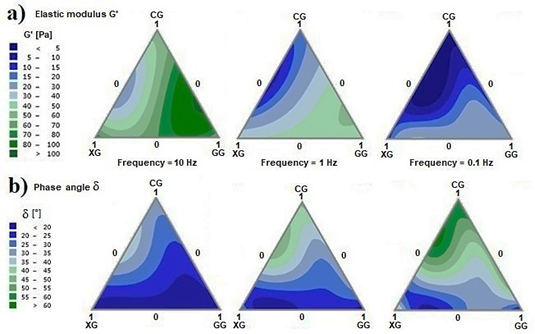
Source: the authors.
Figure 6 Plots of dynamic moduli of CG-XG-GG ternary mixtures at three frequencies (10, 1 and 0.1 Hz). a) Elastic modulus (G’); b) Phase angle (δ).
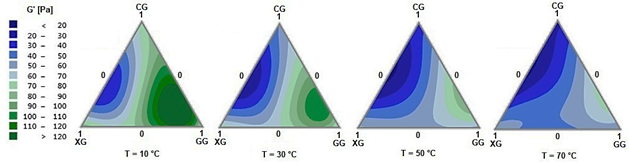
Source: the authors.
Figure 7 Plots of elastic moduli of CG-XG-GG ternary mixtures at different temperatures (10, 30, 50, and 70°C).
By looking at the combined results of Fig. 7 and 8, it is evident that at a lower value of the modulus G’, the gum mixture improves temperature stability. A more solid behavior requires more energy for molecules to move, but once mobilized, it loses its structure and becomes more viscous with small temperature changes. The observation that XG exhibits lower activation energy values compared to GG and CG aligns with the findings in [19], who reports that the higher the pseudoplastic behavior, the lower the temperature dependency. Mixtures of CG-GG are highly temperature sensitive. By incorporating XG into a mixture, more resistant suspensions can be manufactured, such as the mixtures CG-XG that are stable to temperature changes but have low elasticity.
The model for activation energy adjustment as a function of system compositions is shown in Eq. (8) with a correlation coefficient R2 of 0.929.
4. Conclusions
In general, synergistic behaviors have been observed in XG-GG and CG-GG systems, noting that the viscosity value and different rheological parameters, such as shear behavior (pseudoplasticity), in time (thixotropy), and the viscoelastic phenomena of the mixtures is different from the results obtained with the individual components. A direct relationship was found between the results of the Brookfield viscosity and the consistency value (K) of the power law model. For the ternary system CG-XG-GG, pseudoplastic fluid behavior occurs with flow rates (n) greater than 0.60, as well as systems with high pseudoplasticity with flow rates lower than 0.20. It is concluded that the rheological Cross model is a better fit for systems with flow rates greater than 0.40, while the power law model presents the best fit for the experimental flow curves in systems with flow rates lower than 0.40.
With respect to dynamic rheology, binary XG-GG (maximum 50% XG) and ternary (maximum 50% XG and CG greater than 20%) systems have regions with higher elastic behavior that correspond with regions where rheological behavior is less affected over time, with low thixotropy values and stability of the elastic modulus G’ at different frequencies. This can be used to manufacture ternary mixtures with high consistency and low pseudoplasticity. Ternary mixtures with high XG contents are more stable against temperature changes, which is reflected in lower activation energy values.