Introduction
Evolved (andesite to rhyolite) rocks erupted from monogenetic volcanoes have been recognized in all tectonic settings (Németh et al., 2003) and it is described as low-volume (<1 km3) effusive eruptions that occur in a single event or multiple discontinuous pulses in a short period (less than a decade) (Connor and Conway, 2000; Németh, 2010; Kereszturi and Németh, 2012). Such eruptions are fed by dikes whose propagation are perpendicular to the minimum compressional stress (σ3), which is oriented horizontally (Maccaferri et al., 2011). They can form volcanic fields (Németh, 2010; Cañón-Tapia, 2016), where its petrogenesis is explained as individual and spatially separated dikes fed by a common magma supply or reservoir at depth (Smith and Németh, 2017). In this case, evolved compositions are the results of fractionation, mixing and magma contamination processes, that occur due to relatively long residence time during the ascent to the surface (Murcia and Németh, 2020). Evolved monogenetic volcanoes, also occur in the vicinity of polygenetic volcanoes (Torres-Orozco et al., 2017), which are characterized by a complex magma-feeding system with one or more magma reservoirs and a network of dikes and sills (Cashman and Sparks, 2013). This complexity imposes further constraints on the propagation of dikes to the surface, given the variations of the local stress caused by the reservoirs, dikes, and sills (Gudmundsson, 2006; Pansino and Taisne, 2019). Further constraints are expected in the presence of local and regional tectonic structures, as they can modify the state of stress at the crust, which in turn can favor or hinder the propagation of dikes to the surface (van Wyk de Vries and Merle, 1998; Valentine and Krogh, 2006).
In the central part of the Colombian Central Cordillera (Figure 1 A) is located the Tapias dome (4.467706, -75.363457, 2525 masl), a monogenetic volcano situated ~5 km SE of the Holocene Cerro Machín composite volcano (4.486817, -75.386178, 2750 masl; Figure 1 B and 1C). Both volcanoes are built over Permo- Triasic to Jurassic rocks from the Cajamarca Complex, characterized by a package of orthogneiss, phyllites, quartzites, greenschist, and graphite schists (Vargas et al., 2005; Villagómez et al., 2011; Blanco-Quintero et al., 2014). They form the southernmost expression of volcanism in the San Diego - Cerro Machín Volcano- Tectonic Province (SCVTP) (Figure 1 B ). The Tapias dome has a diameter of 1.3 km, a height of 500 m, and an area of ~1.29 km2. On the other hand, the Cerro Machín has a 2.4 km open-crater and an intra-crater dome that stands 490 m in height (Piedrahita et al., 2018). The eruptive history of this volcano is well known (Méndez-Fajury, 1989; Cepeda et al., 1995; Thouret et al., 1995; Rueda, 2005; Murcia et al., 2010), which is characterized by producing dacitic Plinian and Vulcanian eruptions, with the first one occurring 47,000 years BP (Méndez, 2001) the last one only around 900 years BP (Rueda, 2005; Laeger et al., 2013; Piedrahita et al., 2018). The area is influenced by two main dextral strike-slip fault systems (Figure 1 C ), the Otú-Pericos (NE) fault to the East and the Ibagué (ENE) fault to the Southeast (Osorio et al., 2008; Rozo-Gómez, 2012; Gómez-Díaz and Mariño-Arias, 2020).
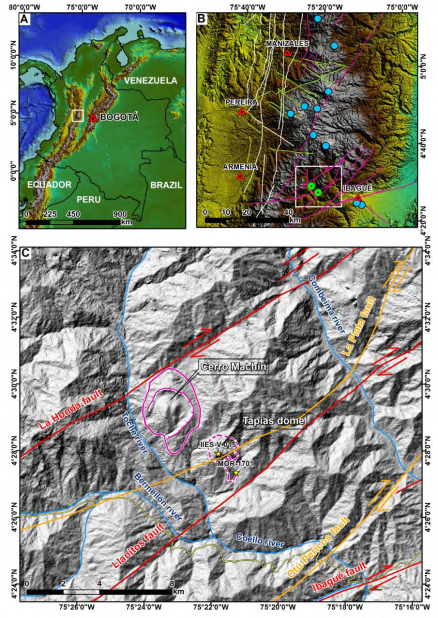
Figure 1 Localization map. A. Physical map of Colombia, white square marks the area of the San Diego - Cerro Machín Volcano- Tectonic Province (SMVTP), located on the central part of the Central Cordillera. B. Detailed map of the SMVTP, blue circles mark the location of the main volcanoes of the province. White lines mark reverse faults, pink lines mark strike-slip faults and green lines mark normal faults. The area of Cerro Machín volcano and Tapias dome is identified with a quite square. C. DEM of the study area. Faults marked in red are part of the Ibagué fault system. Faults marked in orange are part of the Otú-Pericos fault, the fault traces are taken from Cuéllar-Cárdenas et al. (2014) .
In this work, we characterize the Tapias dome through new petrography, mineral and isotopic chemistry, and a K/Ar radiogenic age, together with published whole-rock chemistry. Then, we present geothermobarometric calculations and compare the results with available data from the Cerro Machín volcano. This allowed us to show the link between both volcanic edifices. Finally, we evaluate the volcanism from a tectonic context to provide insights into the interplay between tectonics and magmatism in the area.
Geologic framework
The Plio-Quaternary SCVTP (Figure 1) is a 140 km long magmatic-tectonic segment, located in the axis of the Central Cordillera, in the northernmost part of the Colombian volcanic arc (Martínez et al., 2014). It is the result of the eastern oblique subduction of the Nazca plate below the South American plate, and the collision of the Panamá-Chocó Block (van der Hilst and Mann, 1994; Jaramillo et al., 2019; Mora-Páez et al., 2019). Currently, the SCVTP is located ~280 km from the trench, with crustal thickness reaching up to 52 km below the Cerro Machín (Yarce et al., 2014; Poveda et al., 2015, 2018). At a regional scale, three main types of fault systems have been recognized in the SCVTP (Figure 1 B ). To the west of the Central Cordillera, a group of N-S reverse faults such as the Cauca-Almaguer, Silvia-Pijao, and San Jeronimo (Maya and Gonzalez, 1995) are identified. Across the Central Cordillera, representing regional shear zones, there are the NE-trending right-lateral strike-slip faults such as the Palestina, Ibagué, and Otú-Pericos (Paris et al., 2000; Osorio et al., 2008; Gómez-Díaz and Mariño- Arias, 2020). Towards the middle part of the SCVTP, a group of normal faults such as the Villamaria-Termales (NW-SE) and Santa Rosa (N70E) is present (Thouret et al., 1990; Bohórquez et al., 2005; Mejía-Toro, 2012). This tectonic framework and the systematic inversion of double couple focal mechanisms of shallow earthquakes in the area allowed Cortés et al. (2005) to determine a compressive stress tensor where the principal stress (σ1) is oriented NW-SE within a predominantly strike-slip regime.
Cerro Machín is a Holocene polygenetic volcano whose current edifice was formed from successive Plinian and Vulcanian eruptions (at least six eruptions during the last 5000 years), with magmatic and phreatomagmatic deposits (Rueda, 2005; Piedrahita et al., 2018). Within the products of the volcano, it has been recognized a lava dome (the most recent eruption 900 B.P.) (Laeger et al., 2013; Piedrahita et al., 2018) and PDCs of consistent dacitic composition (59.01-65.99 wt. % SiO2) (Rueda, 2005; Laeger et al., 2013; Errázuriz-Henao et al., 2019). On the other hand, the Tapias dome has not been previously studied, appearing only in the cartography of the area (without any compositional data) defined as an andesitic dome (Mosquera et al., 1982).
From a tectonic context, two main models have been proposed to explain the emplacement of Cerro Machín.
Initially, Mosquera et al. (1982) and Cepeda et al. (1995) proposed that the volcano was emplaced in the intersection of the Cajamarca and Machín faults (see Figure 1 in Piedrahita et al., 2018). However, neither the work of Mosquera et al. (1982) nor Cepeda et al. (1995) clearly describes the location of the Machín fault. In addition, no evidence for the Machín fault using remote sensing studies on the area was observed (Gómez-Díaz and Mariño-Arias, 2020). Another model was proposed by Rueda (2005) , explaining the volcano’s emplacement as the result of a pull-apart structure generated by transcurrent faults (Cajamarca and Salento) with lateral displacement and a high deep angle. Yet, neither of these models explains the emplacement of the Tapias dome and does not take into consideration the two major tectonic systems in the area, which are the right-lateral strike-slip faults Otú-Pericos (NE) and Ibagué (ENE/70° NW), both crossing each other obliquely SE from Cerro Machín and Tapias, which generates a zone of tectonic stress accumulation (Bohórquez et al., 2005; Montes et al., 2005). These faults represent transversal shear zones in the Central Cordillera (Diederix et al., 2006), and accommodate the deformation by developing associated minor synthetic and antithetic faults (Peacock and Sanderson, 1996; Martel, 1997; Kim et al., 2004). In the study area, these faults are recognized as La Plata fault for the Otú-Pericos system, and El Filtro, La Honda, and Llanitos, among others, for the Ibagué system (Figure 1C), as mapped by Cuéllar- Cárdenas et al. (2014).
Methodology
In this work, we present data from two samples from Tapias dome. The Sample IIES-V-O15 (4°27’55.04” N, 75°21’47.43” W, 2160 masl; Figure 1 C) was collected by us during a field campaign in 2019. During fieldwork, we follow the mapping of the dome performed by Mosquera et al. (1982) to find outcrops of fresh rock, from which we collected one rock without noticeable alterations. The sample IIES-V-015 was used for petrographic and mineral chemistry analyses, as well that for radiogenic K/Ar dating. The sample MOR-1701 (4°27’20.97” N, 75°21’15.77” W; Figure 1 C ) was initially reported in the supplementary material of Errázuriz-Henao et al. (2019) as part of Cerro Machín, but it was collected at Tapias dome. From this sample, we use the whole-rock geochemistry data published by Errázuriz-Henao et al. (2019) and present new isotopic data.
Petrography
A thin section from sample IIES-V-015 was made in the Teclab laboratories (Colombia) and their polishing and the application of conductive carbon coating (15 nm) was done at the sample preparation laboratory in the Earth Observatory of Singapore. Conventional petrographic descriptions and point counting (646 in total) were performed, using a Nikon Eclipse 50i microscope from the Mineral Deposits Laboratory at Universidad de Caldas, Colombia. Following González (2008) , we defined phenocrysts (>0.5 mm), microphenocrysts (0.5 - 0.05 mm), and microlites (˂0.05 mm). The groundmass was defined by glass and microlites.
Mineral and Isotopic chemistry
We performed in situ quantitative analysis (45) in plagioclase, amphibole, and biotite phenocrysts and microphenocrysts, using a JXA-8530F Field Emission Electron Probe X-ray Micro-Analyser (FE-EPMA) equipped with 5 wavelength-dispersive spectrometers, at the Facility for Analysis, Characterization, Testing, and Simulation (FACTS) in Nanyang Technological University (NTU), Singapore. Point analyses were acquired using a focused beam at a probe current of 20 nA and an accelerating voltage of 15 kV. The current was reduced to 10 nA and a defocused beam diameter of 3 was used for the analysis of plagioclase, to reduce beam dosage and resultant sodium signal decay. Results were quantified using well-characterized natural and synthetic external calibration standards and a ZAF matrix correction procedure. Oxygen content was assumed from cation abundance, with all iron present as Fe2+. Error on repeat analysis of standard reference materials was <1% of measured values. Kα X-ray lines were monitored for 20 - 60 s for each element, depending on expected concentrations except for Na Kα which was monitored for only 10 s. Background measurements were performed on either side of each peak position for combined counting times equaling the corresponding peak counting times. Measured peak and background positions were found to be free of interferences within the sample and standard matrices. The obtained results were processed and classified using the GCDkit 4,1 (Janoušek et al., 2006), CFU, and CFU-PINGU software (Cortés, 2022). To determine the type of amphibole present we used the Leake et al. (1997) classification, which uses cations per formula unit for the amphibole equation AB2 C5 T8 O22 (OH)2. Some plagioclase and biotite analyses show low values of total oxides; however, these were used with caution. The methodology for isotopic analysis is described in Errázuriz-Henao et al. (2019) .
Radiometric K/Ar dating
Fresh glassy groundmass fragments (<1 mm) from the collected sample were analyzed in ActLabs (Canada) using the following procedure: aliquots of the samples were weighted into an Al container, loaded into the sample system of extraction unit, degassed at ~100°C for two days to remove the surface gases. Argon was extracted from the sample in a double vacuum furnace at 1700°C. The determination of radiogenic argon content was carried out twice on an MI-1201 IG mass spectrometer by isotope dilution method with 38Ar as a spike, which was introduced to the sample system before each extraction. The extracted gases were cleaned up in a two steps purification system. Then pure Ar was introduced into a custom-built magnetic sector mass spectrometer (Reynolds type). Two globally accepted standards (P-207 Muscovite and 1/65 “Asia” rhyolite matrix) were measured for 38Ar spike calibration. For age calculations the international values of constants were used as follows: λK = 0.581 × 10−10 y−1, λβ- = 4.962 × 10−10 y−1, 40K = 0.01167 (аt.%).
Results
Petrography
Macroscopically the Tapias dome displays a porphyritic texture with crystals of plagioclase, amphibole, and biotite (up to 1 cm in diameter), embedded in a glassy light grey groundmass. At a microscopic level, it is observed a prevalent porphyritic texture with subhedral - euhedral phenocrysts and microphenocrysts of plagioclase (0.1 - 2.5 mm, 27 vol.%), amphibole (0.2 - 2 mm, 16 vol.%), biotite (0.2 - 1.8 mm, 2 vol.%), within a glassy groundmass (51 vol.%) with microlites of plagioclase and amphibole (Figure 2). Microphenocrysts of anhedral quartz (up to 0.8 mm, 1 vol.%) and accessory euhedral Fe-Ti (<0.1 mm, 1 vol.%) oxides are also observed. Glomerocrysts of amphibole, plagioclase, and amphibole-biotite also appear, although they are scarce. Plagioclase phenocrysts (Figures 2 A and 2B ) display prismatic habit, inclusions of apatite and amphibole, and diverse zonation patterns: Carlsbad, albite-type, patchy, and oscillatory. A few plagioclase crystals also display well-marked rims (light grey), some of which show dissolution features. This is also observed in the light grey zone of plagioclase phenocrysts with Carlsbad twinning. Amphibole crystals (Figures 2 C and 2D) appear as prismatic and tabular, some with plagioclase inclusions. Two main populations are recognized. Type I amphiboles are crystals without zonation of brown and olive-green colors, whereas type II amphiboles are mostly brown with a marked yellowish rim. Both types of amphiboles are common as phenocrysts and microphenocrysts, although type II appears in relatively larger proportions than type I amphiboles. Biotite phenocrysts (Figure 2 E ) are characterized by their laminar and tabular habit and brown coloration. Some of the phenocrysts display very narrow yellowish rims, and some also display apatite inclusions. Quartz microphenocrysts (Figure 2 F ) are rounded and sometimes fractured.
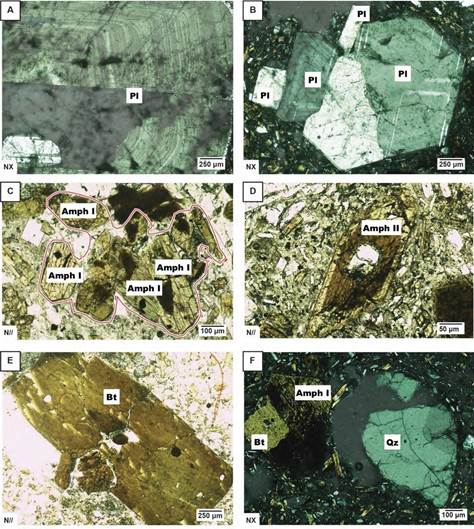
Figure 2 Photomicrographs from Tapias dome (sample IIES-V-015). A. Plagioclase (Pl) phenocryst with oscillatory zonation. B. Plagioclase glomerocrysts, with crystal displaying oscillatory and albite-type zonation. C. Glomerocrysts and phenocrysts of amphibole type I (Amph I) crystals. D. Amphibole type II (Amph II) phenocryst, displaying zonation on its rim. E. Biotite phenocryst. F. Phenocryst of quartz, amphibole type I and biotite.
Mineral chemistry
Table 1 shows the data for the in-situ analysis of the plagioclase, amphibole, and biotite phases. The plagioclase (Figure 3 A ) is classified as andesine (An28-48), and its compositional range falls within the range displayed by-products of the Cerro Machín, which include rocks from the Plinian and Vulcanian eruptions, as well as rocks from the intra-crater dome (Laeger et al., 2013; Regnier, 2015). Likewise, the Mg-rich composition of the biotite crystals (Figure 3B) (Foster, 1960) is observed in both volcanoes. Both amphibole populations observed in the petrography are considered calcic amphiboles (Leake et al., 1997) a (Figure 3 C ), which are also the composition displayed by amphiboles from the explosive and effusive deposit of the Cerro Machín. To better constrain the compositional difference between the two types of amphiboles, we use a binary compositional diagram (Figure 3 D ), which shows that the composition of type I amphiboles is the same composition of the core from the type II (10.20 - 13.10 wt.% MgO). We also notice how the rim from the type II amphiboles is relatively richer in MgO (16.07 - 16.65 wt. %) and hence indicates an inverse zonation. The same behavior is observed in the amphiboles from the intra-crater dome of the Cerro Machín (Laeger et al., 2013).
Table 1 Mineral analyses for the main mineral phases of Tapias dome (sample IIESV-015).
Mineral | Plagioclase | ||||||||||||||||
---|---|---|---|---|---|---|---|---|---|---|---|---|---|---|---|---|---|
Code | C9-b1 | C9-b8 | C9-b9 | C10-a2 | C10-a3 | C10-a4 | C10-a5 | C10-a6 | C10-a7 | C10-a8 | C10-a9 | C10-a10 | C10-a11 | C10-a12 | C10-a13 | ||
SiO2 | 53.87 | 57.64 | 57.27 | 57.03 | 57.40 | 57.84 | 57.96 | 58.41 | 57.36 | 57.84 | 57.96 | 57.74 | 58.20 | 59.04 | 59.44 | ||
Al2O3 | 26.85 | 23.51 | 23.76 | 23.32 | 23.77 | 23.27 | 23.34 | 23.48 | 24.04 | 24.03 | 23.86 | 24.48 | 24.19 | 23.48 | 23.52 | ||
FeO | 0.33 | 0.12 | 0.13 | 0.15 | 0.12 | 0.12 | 0.10 | 0.11 | 0.09 | 0.08 | 0.10 | 0.09 | 0.09 | 0.10 | 0.09 | ||
CaO | 9.91 | 6.04 | 6.51 | 6.11 | 6.27 | 6.00 | 5.95 | 5.84 | 6.60 | 6.37 | 6.47 | 6.85 | 6.61 | 5.82 | 5.90 | ||
Na2O | 5.90 | 8.22 | 7.82 | 8.08 | 7.83 | 7.93 | 8.26 | 8.22 | 7.70 | 7.93 | 7.87 | 7.86 | 8.00 | 8.14 | 8.29 | ||
K2O | 0.20 | 0.43 | 0.40 | 0.44 | 0.41 | 0.42 | 0.44 | 0.43 | 0.38 | 0.39 | 0.37 | 0.36 | 0.38 | 0.44 | 0.43 | ||
Total | 97.06 | 95.97 | 95.89 | 95.12 | 95.79 | 95.57 | 96.04 | 96.49 | 96.18 | 96.64 | 96.62 | 97.38 | 97.47 | 97.02 | 97.66 | ||
% An | 47.59 | 28.17 | 30.80 | 28.73 | 29.97 | 28.80 | 27.76 | 27.52 | 31.45 | 30.08 | 30.57 | 31.86 | 30.70 | 27.60 | 27.54 | ||
Cations per formula unit (c.p.f.u.) based on 8 oxygens | |||||||||||||||||
Si | 2.51 | 2.69 | 2.67 | 2.68 | 2.68 | 2.70 | 2.70 | 2.70 | 2.67 | 2.67 | 2.68 | 2.65 | 2.67 | 2.71 | 2.72 | ||
Al | 1.47 | 1.29 | 1.31 | 1.29 | 1.31 | 1.28 | 1.28 | 1.28 | 1.32 | 1.31 | 1.30 | 1.33 | 1.31 | 1.27 | 1.27 | ||
Fe2+ | 0.01 | 0.00 | 0.00 | 0.01 | 0.00 | 0.00 | 0.00 | 0.00 | 0.00 | 0.00 | 0.00 | 0.00 | 0.00 | 0.00 | 0.00 | ||
Ca | 0.49 | 0.30 | 0.33 | 0.31 | 0.31 | 0.30 | 0.30 | 0.29 | 0.33 | 0.32 | 0.32 | 0.34 | 0.33 | 0.29 | 0.29 | ||
Na | 0.53 | 0.74 | 0.71 | 0.74 | 0.71 | 0.72 | 0.75 | 0.74 | 0.69 | 0.71 | 0.71 | 0.70 | 0.71 | 0.73 | 0.73 | ||
K | 0.01 | 0.03 | 0.02 | 0.03 | 0.02 | 0.03 | 0.03 | 0.03 | 0.02 | 0.02 | 0.02 | 0.02 | 0.02 | 0.03 | 0.02 | ||
Mineral | Plagioclase | ||||||||||||||||
Code | C10-a14 | C10-a15 | C11-a1 | C11-a2 | C11-a3 | C11-a4 | C11-a5 | C11-a6 | C11-a7 | C11-a8 | C11-a9 | C11-a10 | C13-a11 | ||||
SiO2 | 58.45 | 56.20 | 55.65 | 58.24 | 59.86 | 59.16 | 60.67 | 60.14 | 60.78 | 60.03 | 57.96 | 58.88 | 56.75 | ||||
Al2O3 | 24.26 | 26.11 | 27.13 | 25.01 | 24.39 | 24.78 | 24.18 | 24.35 | 24.32 | 24.67 | 24.41 | 23.94 | 23.56 | ||||
FeO | 0.07 | 0.11 | 0.32 | 0.13 | 0.13 | 0.10 | 0.13 | 0.13 | 0.14 | 0.16 | 0.12 | 0.10 | 0.14 | ||||
CaO | 6.51 | 8.59 | 9.80 | 7.55 | 6.53 | 7.11 | 6.10 | 6.48 | 6.12 | 6.55 | 6.76 | 6.14 | 6.44 | ||||
Na2O | 7.73 | 6.71 | 5.81 | 7.20 | 7.87 | 7.52 | 8.22 | 7.75 | 7.96 | 7.63 | 7.77 | 8.06 | 7.80 | ||||
K2O | 0.37 | 0.27 | 0.21 | 0.39 | 0.44 | 0.38 | 0.45 | 0.40 | 0.43 | 0.38 | 0.39 | 0.44 | 0.42 | ||||
Total | 97.39 | 97.99 | 98.92 | 98.53 | 99.23 | 99.05 | 99.77 | 99.24 | 99.75 | 99.42 | 97.40 | 97.56 | 95.10 | ||||
% An | 31.08 | 40.80 | 47.65 | 35.89 | 30.67 | 33.58 | 28.35 | 30.90 | 29.10 | 31.49 | 31.76 | 28.88 | 30.60 | ||||
Cations per formula unit (c.p.f.u.) based on 8 oxygens | |||||||||||||||||
Si | 2.68 | 2.58 | 2.53 | 2.64 | 2.69 | 2.67 | 2.71 | 2.70 | 2.71 | 2.69 | 2.66 | 2.69 | 2.67 | ||||
Al | 1.31 | 1.41 | 1.45 | 1.34 | 1.29 | 1.32 | 1.27 | 1.29 | 1.28 | 1.30 | 1.32 | 1.29 | 1.31 | ||||
Fe2+ | 0.00 | 0.00 | 0.01 | 0.01 | 0.00 | 0.00 | 0.00 | 0.00 | 0.01 | 0.01 | 0.00 | 0.00 | 0.01 | ||||
Ca | 0.32 | 0.42 | 0.48 | 0.37 | 0.31 | 0.34 | 0.29 | 0.31 | 0.29 | 0.31 | 0.33 | 0.30 | 0.32 | ||||
Na | 0.69 | 0.60 | 0.51 | 0.63 | 0.69 | 0.66 | 0.71 | 0.67 | 0.69 | 0.66 | 0.69 | 0.71 | 0.71 | ||||
Mineral | Amphibole | Biotite | |||||||||||||||
Code | C11-b1 | C11-b2 | C11-c1 | C11-c2 | C12-a1 | C12-a2 | C12-a3 | C12-a4 | C12-a5 | C12-c0 | C14-a2 | C14-a4 | C14-a5 | C14-a7 | C2-b | C6-a | C7-b |
Type | I-core | I-core | II-rim | II-core | II-core | II-rim | II-core | II-core | II-rim | II-core | II-rim | II-core | II-core | II-rim | |||
SiO2 | 42.26 | 42.06 | 42.78 | 42.66 | 41.34 | 42.87 | 40.77 | 43.77 | 43.54 | 42.14 | 42.82 | 42.98 | 41.55 | 43.70 | 30.13 | 33.39 | 32.00 |
TiO2 | 1.33 | 1.34 | 2.44 | 1.28 | 1.41 | 2.05 | 1.90 | 1.21 | 2.15 | 1.21 | 2.54 | 1.25 | 1.29 | 2.06 | 3.52 | 3.77 | 3.49 |
Al2O3 | 10.93 | 11.04 | 10.69 | 10.28 | 11.14 | 10.58 | 11.68 | 9.98 | 10.05 | 10.33 | 10.88 | 11.08 | 10.38 | 10.59 | 13.02 | 14.06 | 14.75 |
FeO | 16.63 | 16.77 | 8.53 | 17.08 | 16.95 | 10.30 | 17.00 | 13.86 | 8.81 | 16.83 | 8.90 | 16.27 | 16.91 | 9.37 | 18.33 | 19.15 | 17.89 |
MnO | 0.36 | 0.40 | 0.11 | 0.40 | 0.41 | 0.17 | 0.40 | 0.35 | 0.13 | 0.43 | 0.11 | 0.42 | 0.43 | 0.13 | 0.17 | 0.20 | 0.16 |
MgO | 10.90 | 10.67 | 16.35 | 10.86 | 10.54 | 15.23 | 10.20 | 13.10 | 16.65 | 11.05 | 16.07 | 11.02 | 11.06 | 16.22 | 11.79 | 11.66 | 11.02 |
CaO | 10.87 | 11.01 | 11.00 | 11.14 | 11.08 | 11.02 | 10.74 | 10.72 | 10.67 | 11.10 | 11.07 | 10.93 | 11.05 | 11.01 | - | - | - |
Na2O | 2.06 | 2.08 | 2.46 | 1.98 | 2.05 | 2.35 | 2.33 | 2.11 | 2.50 | 1.96 | 2.51 | 1.99 | 2.06 | 2.43 | 0.67 | 0.53 | 0.64 |
K2O | 0.78 | 0.90 | 0.60 | 0.84 | 0.89 | 0.55 | 0.72 | 0.46 | 0.52 | 0.79 | 0.60 | 0.70 | 0.70 | 0.54 | 8.50 | 8.52 | 7.84 |
P2O5 | 0.01 | 0.01 | 0.06 | 0.03 | 0.03 | 0.04 | 0.03 | 0.01 | 0.02 | 0.00 | 0.03 | 0.01 | 0.02 | 0.04 | - | - | - |
Total | 96.13 | 96.28 | 95.02 | 96.55 | 95.85 | 95.16 | 95.76 | 95.57 | 95.03 | 95.84 | 95.54 | 96.65 | 95.45 | 96.08 | 86.12 | 91.28 | 87.82 |
Cations per formula unit (c.p.f.u.) based on 13 cations | c.p.f.u. based on 22 oxygens | ||||||||||||||||
TSi | 6.34 | 6.33 | 6.26 | 6.41 | 6.27 | 6.31 | 6.18 | 6.48 | 6.34 | 6.36 | 6.25 | 6.39 | 6.30 | 6.32 | 5.19 | 5.37 | 5.31 |
TAlIV | 1.66 | 1.67 | 1.74 | 1.59 | 1.73 | 1.69 | 1.82 | 1.52 | 1.66 | 1.64 | 1.75 | 1.61 | 1.70 | 1.68 | 2.64 | 2.63 | 5.69 |
CAlVI | 0.28 | 0.29 | 0.11 | 0.23 | 0.26 | 0.14 | 0.27 | 0.22 | 0.06 | 0.20 | 0.12 | 0.34 | 0.15 | 0.13 | 0.00 | 0.03 | 0.20 |
CTi | 0.15 | 0.15 | 0.27 | 0.14 | 0.16 | 0.23 | 0.22 | 0.13 | 0.24 | 0.14 | 0.28 | 0.14 | 0.15 | 0.22 | 0.46 | 0.46 | 0.44 |
CFe3+ | 0.83 | 0.74 | 0.80 | 0.73 | 0.76 | 0.82 | 0.78 | 0.94 | 0.98 | 0.85 | 0.76 | 0.79 | 0.91 | 0.89 | - | - | - |
CMg | 2.44 | 2.39 | 3.57 | 2.43 | 2.38 | 3.34 | 2.31 | 2.89 | 3.61 | 2.49 | 3.50 | 2.44 | 2.50 | 3.50 | 3.03 | 2.80 | 2.73 |
CFe2+ | 1.26 | 1.37 | 0.25 | 1.41 | 1.38 | 0.45 | 1.38 | 0.77 | 0.09 | 1.27 | 0.33 | 1.23 | 1.24 | 0.25 | 2.64 | 2.57 | 2.48 |
CMn | 0.00 | 0.05 | 0.00 | 0.05 | 0.05 | 0.02 | 0.05 | 0.00 | 0.02 | 0.05 | 0.01 | 0.00 | 0.00 | 0.00 | 0.02 | 0.03 | 0.02 |
BCa | 1.75 | 1.77 | 1.72 | 1.79 | 1.80 | 1.74 | 1.75 | 1.70 | 1.66 | 1.79 | 1.73 | 1.74 | 1.79 | 1.71 | - | - | - |
BNa | 0.25 | 0.23 | 0.28 | 0.21 | 0.20 | 0.26 | 0.25 | 0.30 | 0.34 | 0.21 | 0.27 | 0.26 | 0.21 | 0.29 | - | - | - |
ANa | 0.35 | 0.38 | 0.42 | 0.37 | 0.40 | 0.41 | 0.43 | 0.31 | 0.37 | 0.37 | 0.44 | 0.32 | 0.40 | 0.39 | 0.23 | 0.17 | 0.21 |
AK | 0.15 | 0.17 | 0.11 | 0.16 | 0.17 | 0.10 | 0.14 | 0.09 | 0.10 | 0.15 | 0.11 | 0.13 | 0.14 | 0.10 | 1.87 | 1.75 | 1.66 |
The superscript T, C, B and A indicate the element position in the amphibole crystal structure.
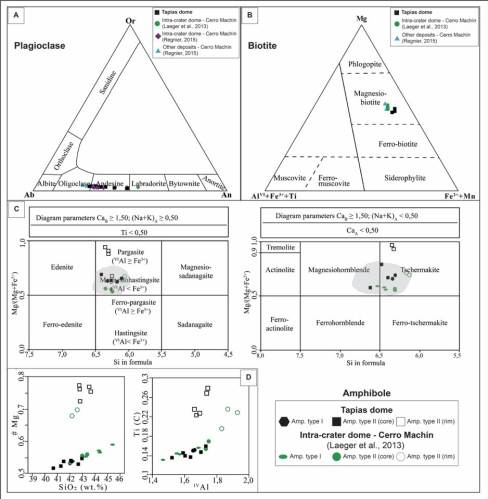
Figure 3 Classification of main mineral phases at Tapias dome (IIES-V-015) and Cerro Machín volcano (CMV). A. Plagioclase composition (Rahman and MacKenzie, 1969). B. Biotite classification (Foster, 1960). C. Amphibole classification (Leake et al.,1997). D. Amphibole binary diagrams of SiO2 vs #Mg and IVAl vs Ti(C).
Whole-rock chemistry and radiogenic isotopes
The concentration of major element oxides, trace elements, and radiogenic ratios are reported by Errázuriz-Henao et al. (2019) in their supplementary material (sample MOR-1701). For the same sample, in this work (Table 2) we report new isotopic data.
The Tapias dome is classified as dacitic with medium-K calc-alkaline affinity (66.59 wt. % SiO2, 2.00 wt. % K2O; Figure 4), which are within the compositions reported for products of the Cerro Machín that have a media of 65.04 wt. % SiO2 and 2.12 wt. % K2O (Cepeda et al., 1995; Rueda, 2005; Laeger et al., 2013; Regnier, 2015; Errázuriz-Henao et al., 2019). To identify the geochemical characteristic of the magmatic source, we consider ratio-ratio plots of highly incompatible elements like Ba/Th vs Ba/Ce (Rollinson, 1993). Figure 5 A shows that the Th/Ce ratio of the samples from both volcanoes seems to follow the same trend. Likewise, rocks from both volcanoes show similar patterns for other trace elements (Figures 5 B and 5C); at the REE diagram normalized to chondrite, the rocks display relative enrichment of the LREE compared with the HREE ((La/Sm)N = 2.9 - 4.3; (Gd/Yb)N = 2.3 - 2.9), whereas the incompatible multi-elements diagram shows decoupling between LILE and HFS elements, with LILE being enriched compared to N-MORB. The rocks also show positive anomalies of Ba, Pb, Sr, and Nd (on a smaller scale), pronounced negative anomalies of Nb, Ta, and P, and minor anomalies of Ti. Eu/Eu* varies between 0.87 and 0.93.
Figure 6 shows the radiogenic isotopes ratios of the Tapias dome compared to data from the Cajamarca Complex (Cavell, 2020) and the Cerro Machín, Santa Isabel, Santa Rosa, and volcanoes (Errázuriz-Henao et al., 2019; Cavell, 2020). To the best of our knowledge, are the closest volcanoes (~25, 34 - 37 km) with known isotopic data. It can be observed how rocks from both Tapias and Cerro Machín plot close to each other and are distinctively different from Nevado del Tolima, Santa Rosa and Santa Isabel volcanoes. Tapias dome shows a higher ratio of both 143Nd/144Nd and 176Hf/177Hf when compared to Cerro Machín, whereas the latter shows a higher 87Sr/86Sr ratio (Figures 6 A and 6B). The inset in both figures shows the isotopic ratios for the Cajamarca Complex (Cavell, 2020), which have noticeably higher ratios of 87Sr/86Sr compared to the volcanoes in the area.
Table 2 Isotopic compositions sample MOR-1701.
Isotope | Value |
---|---|
87Sr/86Sr | 0.704907 |
206Pb/204Pb | 18.960178 |
207Pb/204Pb | 15.643066 |
208Pb/204Pb | 38.787379 |
143Nd/144Nd | 0.512712 |
176Hf/177Hf | 0.282906 |
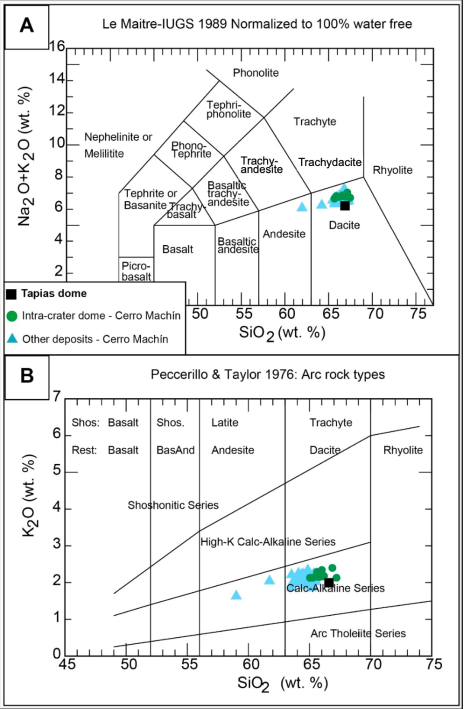
Figure 4 Whole-rock composition of sample MOR17-01 from Tapias (Errázuriz-Henao et al., 2019) dome and Cerro Machín volcano rocks. A. TAS diagram. B. Arc rock types. Blue triangles refer to data from other deposits of Cerro Machín (Cepeda et al., 1995; Rueda, 2005; Laeger et al., 2013; Regnier, 2015; Errázuriz-Henao et al., 2019).
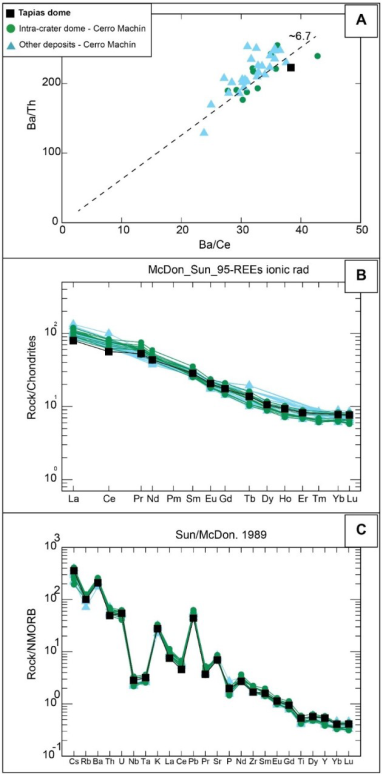
Figure 5 Whole-rock trace element behavior of sample MOR-1701 (Errázuriz-Henao et al., 2019) from Tapias dome and Cerro Machín volcano rocks. A. K2O to Rb ratio. B. REE diagram. C. Multielement diagram. Blue triangles refer to data from other deposits of Cerro Machín volcano (Rueda, 2005; Laeger et al., 2013; Regnier, 2015; Errázuriz-Henao et al., 2019).
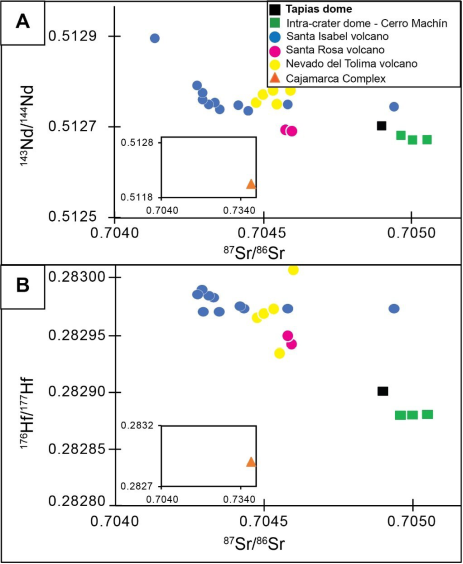
Figure 6 Isotopic compositions for rocks of Tapias dome, compared with Cerro Machín, Santa Isabel (Errázuriz-Henao et al., 2019), Santa Rosa (Cavell, 2020) and Nevado del Tolima (Errázuriz-Henao et al., 2021) volcanoes, and the Cajamarca Complex (inset) (Cavell, 2020). A. 143Nd/144Nd vs 87Sr/86Sr; B. 176Hf/177Hf vs 87Sr/86Sr.
Geochronology
The K/Ar analysis obtained for the Tapias dome yielded an age of 95 ± 0.05 ka (calculated errors are 2σ). Given that it was obtained from the groundmass, it represents the age for the dome emplacement. This age is ~48 ka older than the Boquerón pyroclastic density current (~47 ka), whose rocks are the product of one of the earliest recognized eruptions of Cerro Machín (Méndez, 2001).
Discussion
Crystallization history and amphibole geothermobarometry of Tapias dome
The textures displayed by the Tapias dome reveal a history of magma fractionation and mixing. The presence and composition of mostly euhedral and large phenocrysts of plagioclase, amphibole, biotite, and quartz, imply a prolonged residence at the magma reservoir, where effective fractionation of mafic phases (e.g., olivine, clinopyroxene) permitted the crystallization and growth of minerals with more felsic affinity (Rutherford and Devine, 2003; Holtz et al., 2004). The groundmass microlites represent the last crystallization event before or during the eruption (Hammer et al., 1999; Cashman and Blundy, 2000). However, disequilibrium features such as plagioclase complex zonation patterns (e.g. oscillatory) (Tepley et al., 1999) and the inverse zonation of the type II amphiboles, whose rims are enriched in Mg, imply the influx of mafic magma into the magma reservoir and hence magma mixing (Pallister et al., 1992; Streck et al., 2007).
Given that the two populations of amphibole show equilibrium and disequilibrium features, we use their composition to estimate the conditions of temperature (oC), pressure (GPa), the water content of the melt (wt. % H2O) (Ridolfi and Renzulli, 2012), and oxygen fugacity (log fO 2 ) (Ridolfi et al., 2010), at which they crystallized. This allows us to constrain the depths at which amphibole crystallization occurred and hence provide insights into the magma plumbing system. Table 3 shows the data obtained in our geothermobarometric calculations. It is observed that type I amphiboles crystallized between 871 - 875°C, at 0.27 GPa, with water content in the melt of 7.O wt. % H2O (Figures 7 A and 7B), which is similar to the water content from Cerro Machín volcano (Laeger et al., 2013). By assuming a specific gravity of 2.70 g/ cm3 for the continental crust (Ridolfi et al., 2010), we determine that type I amphiboles crystallize between 10.2 - 10.4 km (Figure 7 C ). As shown in the results, the core of the type II amphiboles is like the compositions of the type I amphiboles, hence their crystallization conditions are also similar, with temperatures between 852 and 920°C, at 0.22 - 0.33 GPa and a meltwater content between 6.0 to 7.5 wt. % H2O (Figure 7 B ); this suggests that crystallization occurred between 8.9 and 12.3 km (Figure 7 C ). On the other hand, the rim of type II amphiboles reflects higher crystallization temperatures (880 - 973°C) and lower water contents of the melt (4.2 - 6.6 wt. % H2O) than their cores, but with similar pressures (0.24 - 0.31 GPa) and hence similar depths (9.1 - 11.8 km) (Figures 7 B and 7C). Regarding the oxygen fugacity (Figure 7 D ), our calculations show similar results for both types of amphiboles, indicating they crystallized in moderate oxidation conditions. These results together with the higher Mg of the rim of the amphibole type II, indicate that it crystallized from a more mafic magma, with relatively lower water content than the one from which the amphibole type I and the core of amphibole type II form. Hence it is assumed that the inverse zonation presented by amphibole type II is the result of the influx of hotter mafic magma into the magma reservoir (8 - 12 km depth) where the residing magma was fractionating (Hawkesworth et al., 2000). This influx of hotter magma is also inferred by 1) the formation of light grey rims on plagioclase pheno-and microphenocrysts, as lighter colors are associated with anorthite-rich compositions (Shcherbakov et al., 2010), and 2) the dissolution features observed in some of the plagioclase crystals that are attributed to the presence of albite rich (XAb >0.6) plagioclase in felsic magmas, as they formed at lower temperatures than the ones required for amphibole crystallization (Rutherford and Devine, 2003; Holtz et al., 2004). Regarding the crystallization of the other mineral phases, Scaillet and Evans (1999) demonstrated that biotite is stable at maximum temperatures of 800°C in water-saturated magma, hence it is possible to assume this as a constrain for the final crystallization temperature for the Tapias dome, as plagioclase and quartz also crystallize at relatively lower temperatures when compare to amphiboles (Scaillet and Evans, 1999; Rutherford and Devine, 2003; Holtz et al., 2004).
Table 3 Estimated crystallization conditions for the amphibole phases identified at Tapias dome.
Ridolfi and Renzulli (2012) | Ridolfi et al . (2010) | Leake et al . (1997) | ||||
---|---|---|---|---|---|---|
Code | Type | T (°C) | P (GPa) | wt. % H2O | LogfO2 | Name |
C11-b1 | I-C | 871.4 ±23.5 | 0.27 ±0.03 | 7.0 ±1.1 | -11.5 ±0.4 | Tschermakite |
C11-b2 | I-C | 874.8 ±23.5 | 0.27 ±0.03 | 7.0 ±1.1 | -11.5 ±0.4 | Magnesiohastingsite |
C12-a1 | II-C | 881.1 ±23.5 | 0.28 ±0.03 | 7.1 ±1.1 | -11.4 ±0.4 | Magnesiohastingsite |
C12-a3 | II-C | 920.5 ±23.5 | 0.33 ±0.04 | 6.3 ±0.9 | -11.4 ±0.4 | Magnesiohastingsite |
C12-a4 | II-C | 886.0 ±23.5 | 0.24 ±0.03 | 6.0 ±0.9 | -11.1 ±0.4 | Tschermakite |
C12-c0 | II-C | 864.7 ±23.5 | 0.24 ±0.03 | 6.9 ±1.0 | -11.5 ±0.4 | Magnesiohastingsite |
C12-c2 | II-C | 852.1 ±23.5 | 0.23 ±0.03 | 6.9 ±1.0 | -11.7 ±0.4 | Magnesiohastingsite |
C14-a2 | II-B | 972.8 ±23.5 | 0.31 ±0.04 | 4.5 ±0.7 | -9.5 ±0.4 | Magnesiohastingsite |
C14-a4 | II-C | 870.0 ±23.5 | 0.28 ±0.03 | 7.2 ±1.1 | -11.6 ±0.4 | Tschermakite |
C14-a5 | II-B | 880.2 ±23.5 | 0.24 ±0.03 | 6.6 ±1.0 | -11.3 ±0.4 | Magnesiohastingsite |
C14-a7 | II-B | 951.8 ±23.5 | 0.29 ±0.03 | 4.8 ±0.7 | -9.6 ±0.4 | Tschermakite |
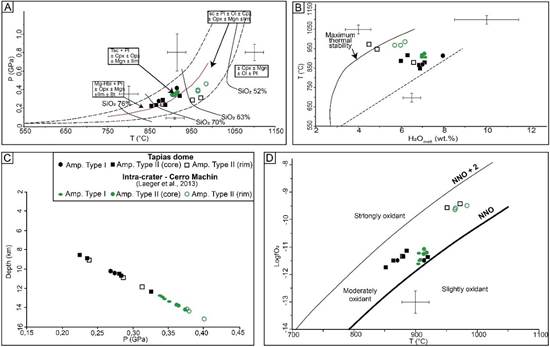
Figure 7 Binary diagrams of geothermobarometric parameters calculated from the Tapias dome and Cerro Machín volcano A. Pressure vs temperature. B. Temperature vs water content on the melt. C. Depth vs pressure. D. log fO2 vs temperature. Error bars represent the expected uncertainty from Ridolfi et al. (2010) . The standard deviation calculated from our data are as follows: T (± 23.5°C), P (± 11.5%), H2O melt (± 15%), log fO 2 (± 0.4 log units).
Tapias dome and Cerro Machín volcano
The dacitic composition with a mid-K calc-alkaline affinity of the rocks from both volcanoes, together with the behavior displayed by the REE and multielement diagrams indicate that these magmas are typical of magmas generated by subduction processes that have been residing and evolving in the continental crust (Wilson, 1989). We propose that Tapias dome was fed by the stagnated magma that currently feeds the Cerro Machín; this is supported by their similar isotopic ratios and the ratio of highly incompatible trace elements (Th/Ce), as these do not vary during fractional crystallization or partial melting (Rollinson, 1993). The compositional characteristics of the magmatic source are described in detail by Errázuriz- Henao et al. (2021). The persistent dacitic composition (65.04 ± 1.31 wt. %SiO2) of the products and the presence of the similar mineral assemblage (andesine, calcic amphibole, Mg-rich biotite, and quartz) in products from Cerro Machín and Tapias, are typical of magmas that have been extensively fractionating at the crust, and thus reinforce the hypothesis that both volcanoes were fed by a common magma. The Tapias dome, as the intracrateric Cerro Machín dome, is the result of effusive eruptions likely associated with degassing during magma ascent linked to bubble connection and therefore permeability in an open system (Fink and Anderson, 2000). We infer that the magma stagnation zone is located between ~9 and 15 km depth, based on our calculations for the amphibole crystallization conditions in Tapias dome and the calculations of Laeger et al. (2013) for Cerro Machín volcano.
Local tectonics and its relationship with the plumbing system
As discussed above, both Tapias dome and Cerro Machín volcano share a common magma; hence questions arise about their spatial separation (~5 km), the time gap (~50 ka) of the volcanic activity, and why such activity moved from Tapias dome to the area where Cerro Machín is located. We infer that these characteristics occur due to tectonic controls at upper levels of the crust, as the rate and angle of subduction of the Nazca plate have remained fairly constant (60 mm/year) in the last 12 Ma (Gustcher et al., 1999). According to the work performed by Cuéllar-Cárdenas et al. (2014) on the Combeima river valley (~8 km NE from Cerro Machín; Figure 8), two structures appear spatially related to the volcanoes studied here; La Plata fault, which can be traced right under the Tapias dome, and La Honda fault, whose trace is observed next to the NW flank of Cerro Machín volcano. These are synthetic structures from the Otú-Pericos and Ibagué fault systems, respectively (Cuéllar-Cárdenas et al., 2014). Hence, we infer that the deformation created by these systems are the ones controlling the emplacement of the volcanoes. Moreover, these authors also identified three consecutive (although not dated) deformation events: An initial strike-slip cinematic deformation caused by the Otú-Pericos system, followed by the development of thrust folding (in a SEE-NWW direction) due to the propagation of the Otú-Pericos fault, and a final right-lateral transport and clockwise rotation of tectonic blocks, caused by the dextral strike-slip deformation cinematics of the Ibagué fault system. We suspect that these sequences of events are linked to the eruptive events represented by Tapias and Cerro Machín (Figure 8). First, it is possible that the La Plata fault (as part of the Otú-Pericos strike-slip system) acted as a path for magma entrapment and propagation to the surface, allowing the emplacement of Tapias dome (Figure 8 B ), similar to observed by Le Corvec et al. (2013) on analytical experiments. After this, the volcanic activity halted during the period of thrust folding, given the reorganization of the stress (σ3 orientates vertically) at the crust (Ferrill et al., 2021), inhibiting magma propagation to the surface (Figure 8 C ) (Valentine and Krogh, 2006; Taherynia et al., 2016). This period of thrust folding is also in agreement with one of the stages of the progressive deformation associated with the development of fold-thrust belts (e.g. the SCVTP) proposed by Ferrill et al. (2021), where early contractional deformation in the strike-slip regime, presides contractional deformation in a thrust-faulting stress regime. Such type of deformation can occur under local to subregional strike-slip conditions. Hence it is possible that in this area and during this period not only the volcanic activity halted but its locus moved in the SEE-NWW direction (towards the Cerro Machín location), which is the direction of the thrust folding observed by Cuéllar- Cárdenas et al. (2014). Lastly, the deformation caused by the Ibagué fault and its synthetic faults (e.g. La Honda fault) caused extensional structures (e.g. pull-apart) that allow the emplacement and construction of the Cerro Machín volcano edifice (Figure 8 D ). Cuéllar-Cárdenas et al. (2014) not only identified hydrothermal and sulphur manifestations associated with the Honda fault (at the valley of Combeima river), but also that deformation associated with the Ibagué fault system over imposes the deformation related to the Otú-Pericos fault system. Accordingly, it is considered that the Cerro Machín volcano is located within the deformation zone of the Ibagué fault system and that there is a symbiotic relationship between the fault system and the volcano (Montes et al., 2005; Osorio et al., 2008; Rozo-Gómez, 2012).
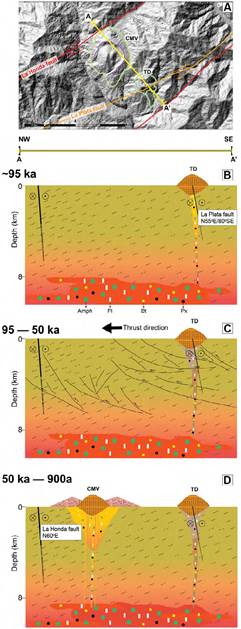
Figure 8 Proposed model for the emplacement of Tapias dome and Cerro Machín volcano. A. The DEM of the area is added as a geographic reference. B. At <95 ka, dike propagation to the surface is aided by the movement of La Plata fault (part of the Otú-Pericos system), allowing the emplacement of Tapias dome. C. Between 95 and 50 ka, thrusting deformation in a SE-NW direction inhibits propagation of magma to the surface. D. Starting at ~50 ka, extensional deformation caused by the Ibagué fault and its synthetic structures (i.e., La Honda fault), facilitate magma ascent and thus prolonged stagnation and therefore the building of Cerro Machín volcano. Green hexagons represent amphiboles crystallising at the magma chamber (phenocrysts and microphenocrysts) and during ascent (microcrystals), likewise black dots represent biotite, white squares represent plagioclase and yellow starts oxides.
The area where Tapias dome and Cerro Machín volcano are located displays numerous lineaments and faults-like structures (Gómez-Díaz and Mariño- Arias, 2020), however, for some of them there is no consensus regarding their name or even their classification as a fault. For example, pull-apart structures have previously been invoked to explain the location of Cerro Machín (Mosquera et al., 1982; Cepeda et al., 1995; Rueda, 2005) and were attributed to deformation caused by the Cajamarca fault. Here we consider that both Cajamarca and La Honda fault are just two different names for the same structure, as their trace seems to follow roughly the same path. However, a discussion of the possible different tectonic faults in the area is beyond the scope of this work. Nevertheless, we consider that our model does explain the coincidence between the sequence of volcanic events and the location of tectonic structures; even if there are no chronological constraints (to the best of our knowledge) for the tectonic deformation events described above so we could establish a direct link.
Conclusions
Tapias dome is a dacitic monogenetic volcano (<95 ± 0.05 ka), characterized by a mineral assemblage of plagioclase (An28-48), amphibole (tschermakite and magnesiohastingsite), biotite, quartz, and Fe-Ti oxides, embedded in a glassy groundmass.
Two amphibole populations are recognized, with and without zonation (High-Mg rims). The core of zoned crystals has the same composition as the unzone ones, indicating an influx of mafic magma into the reservoir.
Geothermobarometric calculations on the amphibole populations in Tapias dome indicates a magmatic stagnation zone at depths between 8.5 and 12.3 km, which would agree with the deepest magma chamber, previously unidentified for the Cerro Machín (10 - 15 km).
Similarities between the rocks of Tapias dome and Cerro Machín volcano suggest that they are fed by the same magma. The rocks are similar in their dacitic composition; their mineral chemistry: andesine plagioclase, Mg-rich biotite, and two populations of amphibole; as well that their isotope ratios (e.g., 87Sr/86Sr: 0.704907 for Tapias dome and 0.704957 to 0.705046 for Cerro Machín volcano).
The spatiotemporal separation (~50 ka) between Tapias dome and Cerro Machín volcano seems to be controlled by the synthetic faults developed to accommodate the deformation of the Otú-Pericos fault (La Plata fault- Tapias dome), which is later over imposed by the deformation of the Ibagué fault system (La Honda fault - Cerro Machín).