Introduction
Ancient sediments are a window into the past and, through the analysis of fossils, isotope fingerprints, geochemical composition, and mineralogy, it is possible to understand the coevolution of the planet with life. Peridotites are mantle rocks commonly exposed in oceanic fracture zones and along low-angle faults related to tectonic extension and crustal thinning at slow-spreading mid-ocean ridges (e.g., Escartín et al., 2003; Mével, 2003; Alt et al., 2007). The oceanic peridotites are subject to an intense activity driven by heat supply provided by the intrusion of mafic magmas or by cooling of the ultramafic lithosphere which produces serpentinization and fluid circulation (Alt et al., 2007). These create a hydrothermal environment that can support vent communities (e.g., Kelley et al., 2001). Oxygen isotope data from the Hess Deep in the Pacific Ocean, indicate that serpentinization of the upper mantle occurs at temperatures of 275-375ºC when seawater penetrated downward during rifting related to the propagation of the Galapagos spreading center (Agrinier et al., 1995; Früh-Green et al., 1996; Alt et al., 2007). However, serpentinization reactions can also take place at relatively low temperatures (0-150ºC) in fluid vent sites where microbial activity has been detected (e.g.,Agrinier et al., 1995; Alt and Shanks, 1998; Kelley et al., 2001, 2005; Alt et al., 2007). Different fine-grained type sediments can be associated with the activity surrounding hydrothermal vent systems hosted by ultramafic rocks exposed in the oceanic fractures and mid-ocean ridges (e.g., Früh- Green et al., 2004; Ludwig et al., 2006). Metalliferous sediments have been associated with hydrothermal systems occurring on various spatial and time scales principally in ocean ridges. The metal enrichment has been attributed to high-temperature hydrothermal sources (Mills and Elderfield, 1995a), but also to a low- temperature hydrothermal activity supported by oxygen isotope studies of nontronite within the sediment (e.g.,McMurtry and Burnett, 1975; McMurtry and Yeh, 1981) or with the transport of particles by bottom currents in deep fracture zones of the East Pacific Ridge (EPR) (Heath and Dymond, 1981). Mills and Elderfield (1995a) documented in the Pacific Ocean the formation of basal metalliferous sediment that overlies igneous crust. These deposits consist of poorly crystalline to X-ray amorphous Fe-Mn-oxyhydroxides, clay minerals, and amorphous silicate phase (Dekov et al., 2010). The oxyhydroxides furthermore are efficient scavengers for rare earth elements (REE) and Yttrium along with others trace elements such as Ni, Co, and Cu (Ruhlin and Owen, 1986; Mitra et al., 1994; Hein et al., 1997; Kuhn et al., 1998). Metalliferous sediments are characterized by a very high Fe and Mn content (on an abiogenic basis), very low Al/(Al + Fe + Mn), high content (on an abiogenic basis) of As, Ba, Be, Bi, Cd, Co, Cu, Mo, Ni, Pb, Sb, Th, U, V, W, Y, Zn, and Zr and depleted in Al (and Ti) relative to pelagic background sediments (Dekov et al., 2010). Their REE distribution patterns are like that of deep seawater and show weak signs of hydrothermal imprint (weak positive Eu anomaly). Metalliferous (hydrothermal) sediments distributed on ridges are associated with the deposition of metal-rich phases from near volcanic emanations (Boström and Peterson, 1969; Slack et al., 2009), and have been linked to the combination of hydrothermal plume fallout of Fe and Mn oxyhydroxide, mass-wasting of sulfide mound debris, and sedimentation of detrital and biogenic particles (Metz et al., 1988; German et al., 1993; Mills et al., 1993; Mills and Elderfield, 1995a; German and Von Damm, 2003).
REE distribution pattern in metalliferous sediments provides valuable information about the elemental source and dissolved oxygen levels (Byrne and Sholkovitz, 1996; Nozaki, 2001; Nozaki and Alibo, 2003). REE patterns vary from fluid to seawater by both scavenging of particles in the water column (Byrne and Sholkovitz, 1996; Nozaki, 2001) and fractionation from the hydrothermal fluids during precipitation (Mitra et al., 1994; Mills and Elderfield, 1995b). The REE behavior in high-temperature seafloor hydrothermal fluids is well characterized (Michard and Albarede, 1986; Klinkhammer et al., 1994). Highly enriched REE concentrations in high-temperature hydrothermal vent fluids up to 10 × 104 times ambient seawater concentrations have been measured in several sites: Trans-Atlantic Geotraverse (TAG) chimney oxides from Mid-Atlantic Ridge (MAR) (Mills and Elderfield, 1995b); Juan de Fuca Ridge (Hinkley and Tatsumoto, 1987), Snake Pit area also called MARK site along MAR at 23°N (Campbell et al., 1988) and East Pacific Rise at 13°N and 21°N (Michard et al., 1983; Michard and Albarede, 1986). Black smoker fluids exhibit a distinct REE pattern with light REE (LREE) enrichment, a large Eu anomaly, and negative or no Ce anomaly (Klinkhammer et al., 1994; Mitra et al., 1994). This pattern is distinct from seawater which has no Eu anomaly, a large Ce anomaly, and heavy REE (HREE) enrichment (Mills and Elderfield, 1995b). According to Klinkhammer et al. (1994), hydrothermal fluids may represent a significant secondary source of REEs (especially Eu) in the oceans. REE-Y patterns are commonly used to trace the origin of metalliferous deposits (Sui and Lasby, 1998). Recently, using REE elements relations Hodel et al. (2018) demonstrate that the Aït Ahmane ultramafic unit of the ca. 760 Ma Bou Azzer ophiolite (Morocco) hosts a fossil black smoker- type hydrothermal system.
In northwest Colombia, at the northern end of the western Andean Cordillera, a set of rocks formed by claystone and mudstone rests on top of the Cerro Matoso peridotites (Castrillón, 2019). The set of rocks was accreted during the Cretaceous and later exposed to weathering conditions. The peridotites developed a nickel laterite profile from which is mined, molten, and processed to produce, in one of the largest lateritic nickel mines worldwide, an alloy of iron and nickel of high purity and low carbon content, for exportation to the international markets, mainly Asia. Recently, Castrillón et al. (2022), using XRD, BSE-EDX, and SEM analyses, described petrographic and mineralogical characteristics of the claystone/mudstone overlying the Cerro Matoso peridotites in the Pit-1, north of the mine. The XRD analysis permitted the identification of siderite as the only carbonate in the samples and petrographic and SEM studies allowed classifying the siderite as a diagenetically altered mineral that are infilling cracks, pores, and pipes acting as a cement. These sediments were identified as deep marine claystone forming mound structures and black mudstone forming tabular beds.
Here, new petrographic and mineralogical data combined with oxygen and carbon isotopes, EPMA, and ICP-MS analyses, including trace elements and REE+Y distribution from the claystone and mudstone on top of the Cerro Matoso peridotites, are presented as novel evidence of the formation of deep-marine sediments hosted by peridotites with serpentinization- related and released fluids from the mantle section of the ancient mid-ocean Pacific ridge. This improves our understanding of the mechanisms involved in the formation of the Cerro Matoso metalliferous sediments.
Regional setting
The Cerro Matoso deposit is in northwest Colombia, about 100 km southeast of Montería in the province of Córdoba, in the northern end of the Western Andean Cordillera. The peridotite body occurs in one of a series of isolated outcrops assigned to the Cauca ophiolite complex of the Early Cretaceous age (Mejia and Durango, 1981). This complex is a relict of oceanic crust originated in the Pacific Ocean, far west of its present position (Pindell and Barrett, 1991; Pindell and Kennan, 2009). The ophiolitic complex of which Cerro Matoso is part was tectonically accreted during the Pre-Andean orogeny along the Romeral Fault System, considered as a major boundary between oceanic crust to the west and continental crust to the east (Barrero, 1974; Meissnar et al., 1976). Migration of theAmerican continent to the west and of the oceanic plate to the east caused the accretion of oceanic segments to the western margin of the continent (Lewis et al., 2006). Probably the emplacement of Cerro Matoso and Planeta Rica peridotites took place during the pre-Andean orogeny (Middle Eocene) and from that moment, the laterization process began (Dueñas and Duque-Caro, 1981; López-Rendón, 1986; Hoyos and Velázquez, 1996). The ultimate exhumation and ultramafic rocks exposition to the supergene weathering conditions was during the Andean orogeny (Figure 1). The ultramafic rocks are surrounded by basalts (Ksvb) (outcropping 30 km to the south), and the sedimentary succession from early to mid-Miocene that includes a transgressive succession consisting of fluvial and shore sandstones (Ciénaga de Oro Formation-Pgoco), followed by marine shales (Porquera Formation-Ngmpc) interpreted to unconformably overlie the oceanic rocks. Finally, marine sediments (Cerrito Formation-Ngmc and Sincelejo Formations-NgQsi) unconformably overlie the Porquera Formation.
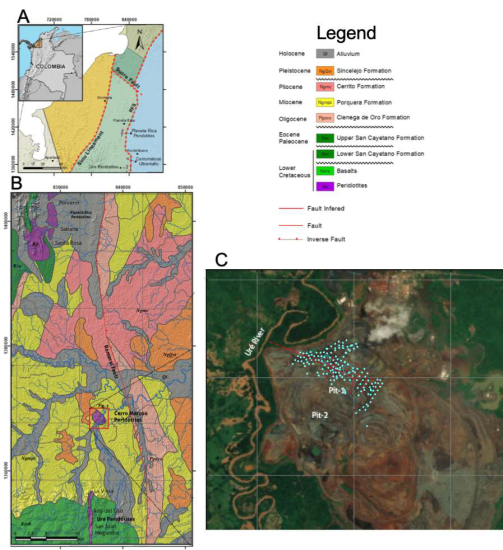
Figure 1 A. Cerro Matoso regional location. B. Cerro Matoso peridotites are unconformably covered by the latest Oligocene to Pleistocene strata, including the Ciénaga de Oro (Pgoco), Porquera (Ngmpc), Cerrito (Ngmc), and Sincelejo (NgQsi) Formations. Modified from the SGC Geological maps 72 and 82 (Pueblo Nuevo and Montelibano) and from Tobón et al. (2020) . C. Cian dots show the sedimentary distribution of the black mudstone succession at Pit-1 with a thickness varying between 10 to 20 m.
Local geology
According to López-Rendón (1986) , lateritization probably started in the late Eocene-early Oligocene, and chemical weathering and erosion continued through Oligocene. The laterite profile and Ni enrichment in Cerro Matoso suggest different episodes of weathering during the Cenozoic (Gleeson et al., 2004). According to López-Rendón (1986), the laterites of Cerro Matoso consist of 5 main horizons from base to top: unaltered peridotite, saprolitized peridotite, saprolite, limonite, and iron hat (gossan) or ¨duricrust¨. Gleeson et al. (2004) describe 2 different alteration profiles at Cerro Matoso, one developed on Pit-1 toward the N sector of the mine and the other on Pit-2 toward the S sector and identified in both pimelite and sepiolite as the major nickel-bearing silicate phases, followed by smectites (saponite) and chlorites (nimite). Seven horizons have been described in the laterite profile of Cerro Matoso at the Pit-1 (Gleeson et al., 2004; Tobón et al., 2020), although four of these units named: ̈black saprolite ̈, ̈ black canga ̈, ̈red laterite ̈, and ̈canga mona ̈ (e.g.,López- Rendón, 1986; Ortiz, 2004; Sumicol, 2002; Gleeson et al., 2004; Tobón et al., 2020) were redefined as deep marine claystone/mudstone by Castrillón (2019) . At south or Pit-2, the peridotite alters to saprolitized peridotite, high and low-magnesium green saprolites, brown saprolite, and high and low iron laterites, without ferricrete or duricrust. The nickel content at Pit-1 overpassed 9% while at Pit-2 did not surpass 4%.
Methodology
A representative set of 34 samples were collected at the 49 Bench level of Pit-1, where crops out the marine sediments (Figure 1B). The sediments are differenced by 1) a mound structure succession composed of fossiliferous claystone and mudstone at the base (Table 1, Figure 2A-2D), and 2) a tabular bed succession formed by orange iron laminated claystone and fossiliferous red claystone (Figure 2E) that lie over black mudstone that contains intraclast of serpentine and red claystone (Figure 2 F-2I). The latter are overlaying listvenites, which also present two varieties (Figure 2 J-2K). The listvenites are capping green saprolites (Figure 2 L). Downwards the laterite profile is completed of altered peridotites (Figure 2 M), peridotites with magnesite veins (Figure 2 N), and peridotites (Figure 2 O).
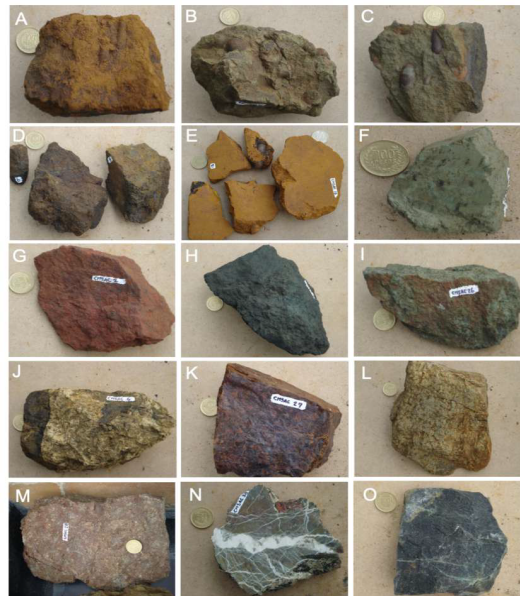
Figure 2 Hand samples collected from the Cerro Matoso deposit at Pit-1. A. Brown oxidized claystone. B-C. Green fossiliferous claystone. D. Fissured brecciated mudstone. E. Fossiliferous red claystone. F. Intraclast of serpentinite. G. Intraclast of red claystone. H. Black mudstone facies II. I. Black mudstone facies I. J. Listvenite variety A is characterized by brecciated textures with high silica/siderite vein content. K. Listvenite variety B is characterized by a purple color. L. Low-Mg green saprolite. M. Saprolitized peridotite. N. Magnesite and silica veins in peridotite. O. Fresh peridotite.
Table 1 Summary of samples collected at Cerro Matoso Bench 49, Pit-1.
Rock name | Sample cm Id* | Total |
---|---|---|
Orange iron laminated claystone | 46 | 1 |
Red claystone | 13 | 1 |
Green Fossiliferous claystone | 10, 21, 22, 23, 24, 25, 32, 33 | 8 |
Fissured brecciated mudstone | 11 | 1 |
Black mudstone | 19, 20, 26 | 3 |
Intraclast | 1, 2, 5, 9, 35 | 5 |
Listvenites | 3, 4, 6, 7, 18, 27 | 6 |
Green saprolite | 12, 34 | 2 |
Saprolitized peridotite | 15, 17, 28, 30 | 4 |
Peridotite | 16, 29 | 2 |
Serpentinized peridotite | 14 | 1 |
Note: *Related with Table 4.
A total of 42 subsamples of the greenish and blackish sediments for analysis were extracted from the internal regions of the original rock sample to avoid contamination. All subsamples were cleaned and subdivided into polished, resin-free rock chips and freshly broken fragments. Petrography was done using the binocular and petrographic microscope Carl ZEISS Primo Star HD/Full Köhler cam, 100x, SF2, from the Instituto de Geología of the Universidad Nacional Autónoma de México. Specimens were examined in hand samples and a microscopic study on transmitted and reflected light was made. Isotopic analysis was done on bulk samples, and samples of siderite concretions. This analysis was accomplished by reacting a subsample (10-48 mg dry weight) with 100% phosphoric acid in a Pyrex vessel at 50°C. The reaction was terminated after 10 days, at which time CO2 was no longer produced, and the reaction was considered completed (Al-Aasm et al., 1990).
The evolved CO2 was used for the calculation of siderite abundance in the subsample and the analysis of oxygen and carbon isotope compositions. Isotope measurements were carried out at the Stable Isotope Laboratory of the Instituto Andaluz de Ciencias de la Tierra (CSIC, Granada, Spain). Isotopic ratios were measured by a Finnigan MAT 251 mass spectrometer. The reproducibility of the analytical procedure was lower than ±0.2‰ for carbonate Fe-Mg. All the samples were compared to a carbon dioxide sample obtained from siderite and calcite standards prepared at the same time. A fractionation factor (αsiderite-CO2) of 1.01006 (Rosenbaum and Sheppard, 1986) was used to back- calculate the isotopic compositions of siderite due that CO2 has a different oxygen isotope composition than siderite, from which the gas was evolved (e.g.,Zhang et al., 2001). Oxygen and carbon isotopes of siderite were reported relative to the standard - Vienna Pee Dee Belemnite (V-PDB). Oxygen data were also converted into SMOW using the Coplen et al. (1983) equation: δ18O‰ (SMOW) = 1.03090 × δ18O‰ (V-PDB) + 30.91 to investigate the relationship with temperature. The isotopic modeling for carbon considered a marine environment with an isotope pool of H2S, CH4 , OH-, HCO3 - and Fe, where CaCO3 and CH4 are products of the isotopic exchange during an electron loss and gain process. The fractionation factor resulting from anaerobic or aerobic oxidation of methane was 1.039 (Whiticar and Faber, 1986; Templeton et al., 2005) and was considered the maximum value of the isotopic fractionation ∆ = 39‰, for product 1 or CaCO3. The residual substrate or product 2 (CH4), takes a ∆2 = 12.3‰ value which represents the highest isotopic depletion in the aqueous medium. An equilibrium constant of kinetic isotope effect KIE = -32 was considered. The fractionation model for δ18O considered O2 and CaCO3 as products 1 and 2 that are generated in the process of electron loss and gain in an open system. The isotopic fractionation ∆ = -31‰ for O2 was suggested by Fu et al. (2015) for hydrothermal systems and for CaCO3 the ∆2 = 10‰ was suggested by Li et al. (2006) . The equations used for the isotopic modeling were:
Δ pool = Δ 2 + f + Δ 1 - Δ 2 × f
f13C = 1 - (δ13C‰(V-PDB)/KIE), KIE = -32
f18O = 1 - (δ18O‰(V-PDB)/KIE), KIE = 10
ln = LN (f)
α = (1000 + KIE)/(1000 + δ18O‰(PDB))
106 T2 = 103 LN (α) - 1.69/2.56
δ18O‰(SMOW) = 1.03090 × δ18O‰(PDB) + 30.91
δ18O‰(V-PDB) = δ18O‰(SMOW) × 0.97002 - 29.98
δ13C‰(V-PDB) = δ13C‰(V-PDB) + (δ13C‰(V- PDB) × 1.95/100)
Oxygen Isotope fractionation = 103 LN (α)
Later, thin sections were critically point-dried and coated with a thin layer of carbon to be analyzed with an electron probe microanalyzer (EPMA) JEOL JXA-8900 XR, at the Laboratorio Universitario de Petrología of the Instituto de Geofísica Ambiental, Universidad Nacional Autónoma de México (UNAM). This equipment allowed us to obtain back-scattered electron (BSE) images, together with X-ray energy dispersive spectroscopy (EDS), qualitative analyses, and wavelength dispersive spectroscopy (WDS) for quantitative analysis of the mineral grains. The conditions used during this analysis were: 20 KeV, a beam diameter of 1 μm, and a counting time of 30 s. The measured conditions were for K, Na, Ba, Ca, Sr, Mn, Mg, and Fe with standards of Guaymas Data. This analysis provides data on the chemical composition of carbonates. The analysis focused on the oxides content of Fe carbonates, magnetite, and clays. The results were normalized for CO2.
Bulk-rock chemical analysis of 10 samples was performed at Gmas Lab. (Bogotá) by x-ray fluorescence in fused LiBO2/Li2B4O7 disks using a BrukerS4- Explorer X-ray fluorescence spectrometer with an Rh-anode X-ray tube as a radiation source. The semi- quantitative and quantitative analysis was carried out using the QUANT-EXPRESS method (fundamental parameters) before calibration of the equipment with certificated USGS standard samples (AGV1, BCR2, DST2, QLO1, G2, and W2A). Additionally, chemical analysis of 5 bulk-rock samples was performed at Activation Laboratories Ltd (Actlabs) in Canada, with the Ultratrace 5 analytical package (Instrumental Neutron Activation Analysis, INAA + Inductively coupled plasma mass spectrometry, ICP-MS) in a Thermo Finnigan-High Resolution mass spectrometer. The detection limits were 0.01% for major elements and 0.5 ppm for trace elements, with a precision of ±5%. The REE concentrations are normalized relative to the composition of the standard Shales (Piper, 1974). Scanning electronic microscope Hitachi-TM-1000 and the wd/ed microanalyzer JXA-8900R, both from the Instituto de Geofísica Ambiental (UNAM), were used to identify the mineral and chemical components of the selected samples. Standards used for calibration of the equipment included pure metals and synthetic and natural minerals (carbonates and sulfates) from the set of standard SPI # 02753-AB serial No. 02-148.
Results
Field relations
Fossiliferous claystone (mound structures): On top of the peridotites rest a mound structure characterized by a massive and discontinuous unit approximately 14 m high and 50 m long. With a predominantly clay grain size, the claystone unit is characterized by its high porosity, and numerous hollow vug and tubular structures with an orientation that is roughly perpendicular to the base of the mound. The tubes are visible (15 m in diameter) and look like forming colonies. Some tubes are infilled with the same material as the substrate while others are infilled with siderite which also works as cement. This succession is laterally in contact with black mudstone. From the top, three facies can be differentiated:
In the upper part, a segment of 3 m thickness is formed of brown-colored claystone (bo-C) facies, which is characterized by an apparent layering, rough surfaces, tube structures (9-25 mm in diameter), intraclast and bioclast content (Figure 3 A). In thin section, the fossil (i.e., gastropods) content is fully replaced by goethite, the principal mineral identified by XRD (Table 2).
Table 2 Hand sample description and XRD results.
Facies name | Hand sample description | Principal minerals XRD (Castrillón, 2019) | Subsample Id | |
---|---|---|---|---|
Tabular beds | Black conglomeratic mudstones II | Fine sand to coarse silt size and friable rock with muddy texture. Dark bluish green (5BG 3/2) and very pale green (10G 8/2) colored. Clayish matrix. Magnetic. | Greenalite, nimite, siderite, goethite, magnetite, chromite, Fe oxide | cm-19 54 19 |
Black conglomeratic mudstone I | Clayish rock mottled in appearance. Green (10G 8/2) to pale green (5G 7/2) colored, alternating with noncontinuous orange, red, and black laminations associated to Fe oxides and manganese. | Greenalite, berthierine, siderite, goethite, manganese oxide, nimite, magnesium sulfate | 26 | |
Serpentine intraclast | Weakly foliated massive hard rock of pale yellowish green in color (10GY 7/2) with phaneritic texture and weakly oriented mafic of very coarse sand size. | Siderite, antigorite, olivine, talc, serpentine, spinel, | 9 | |
Claystone intraclast | Well cemented Claystone. Moderate reddish brown (10R 4/6) and mottled in color. | Greenalite, berthierine, siderite, goethite, nimite, chromite | cm-27 35 | |
Mound structure | Brown oxidized claystone | Well cemented and altered claystone with a low fossiliferous content, very high porosity formed by open conduits, fissures, or burrows, low proportion of intraclast, and bioclast. | Greenalite, goethite | cm-11 23 |
Green fossiliferous claystones | Well cemented fossiliferous claystone, with very high porosity formed by open conduits, fissures, or burrows, low proportion of intraclast, and bioclast. | Berthierine, nimite, siderite, goethite | cm-5 32 49 | |
Fissured brecciated mudstone | Brecciated and fissures (<1mm width) black to dark reddish-brown colored mudstone with fragile and brittle appearance, poorly fossil content. Porosity associated to open conduits, fissures, or burrows. | Siderite, goethite, nontronite | cm-1, 11a |
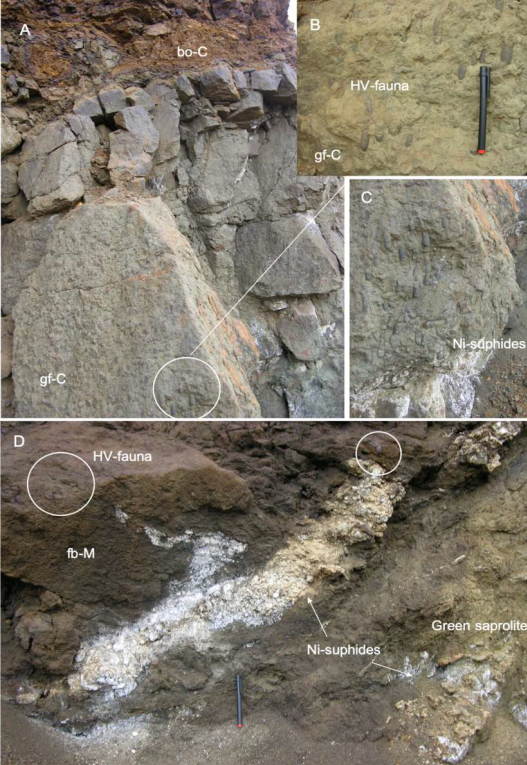
Figure 3 A. Outcrops of brown oxidized claystone (bo-C) overlaying fossiliferous green claystones (gf-C). B-C. Detail of tubeworm fossils and vent fauna forming the gf-C facies. D. Fissured brecciated mudstone (fb-M) with bioturbation overlaying green saprolites and Ni-sulphides (white) in both units.
Downwards throughout a net contact, a 6 m thickness unit corresponds with green fossiliferous claystone (gf-C) facies, which is characterized by the tube structures and the fossil content that include gastropods, bivalve shells, and tubeworms (15-20 mm in diameter). The fauna is densely packed, some are randomly arranged but many are vertical and appear in a living position (Figure 3 B, 2C). Pipes and conduits are common as well as nodules or concretions. In thin section, the green matrix has a low proportion of bioclast and intraclast. Siderite is infilling cracks, pipes, pores, and forms concretions (Figure 4 A-4C). Berthierine and siderite were detected in XRD as the major minerals.
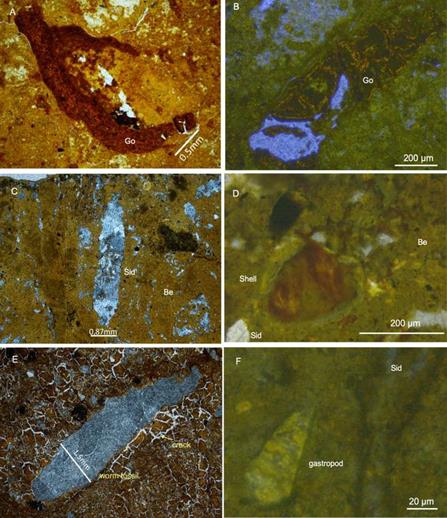
Figure 4 Thin sections. A. Brown oxidized claystone forming by goethite and fossil relict. B. Detail of fossil relic structure. C. Berthierine and siderite forming fossiliferous green claystone facies. Siderite are infilling pores. D. Detail of altered shell fossil into the berthierine matrix. E. Siderite infilling cracks and pores in the fissured brecciated mudstone. F. Gastropod fossil into the fb-M facies.
Throughout a transitional contact, a 3 m thickness unit is described as a fissured brecciated mudstone (fb-M) underlying the gf-C facies. This unit is characterized by its brittle and fragile appearance, with minor tubular and fossil content. White surfaces over the rocks correspond to Ni-sulphide. In thin section, an intensely fractured or fissured muddy matrix predominates where the siderite is filling the fissures as well as the mold and vug porosity. Gastropods fossils can be recognized. This thin unit rest over green saprolites in which also Ni-sulphides are present.
Iron-rich Mudstones (tabular beds): It is a massive, tabular, and continuous carbonate-Fe-bearing mudstone succession of approximately 40 m of thickness in which three facies can be distinguished. In the upper part, a 4 m thickness unit described as brown orange laminated claystone (oil-C) facies is formed by discontinuous hard beds of Fe-rich claystone in thin layers. Siderite, goethite, and magnetite were detected in XRD as the mayor components (Figure 5 A ). Throughout a transitional contact, a 20 m of thickness unit described as black mudstone (BM) facies is underlying the oil-C facies. This coarse-grained appearance unit forming tabular beds usually looks extensive and stratified and can be divided into two facies (BM I and BM II).
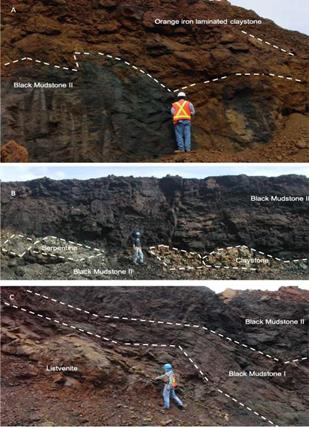
Figure 5 A. Orange iron laminated claystone (oil-C) overlaying black mudstone facies. B. Black mudstone facies reaching locally a 20 m thickness with claystone and serpentine boulder embedded. C. Black mudstone facies overlying listvenites.
The upper facies (BM II) is a blackish unit with a brittle and granular mud-sandy appearance, poor cemented and poorly crystallized oxy-oxyhydroxides in a fine matrix (Figure 5B). The framework is composed of bioclasts and intraclasts in a similar percentage, the bioclasts observed are shell fragments and the intraclast are fragments of serpentinites (Figure 6 B ). In thin section, intraclasts of claystone presents locally wavy laminated structures that have been replaced by goethite and oxides, giving appearances of bioconstructions with siderite and oxides cementing and replacing old bacterial filaments and channels (Figure 6 A , sample cm-01). Siderite also is present as part of claystone intraclasts. FeO-rich oxides (magnetite) formed abundantly, usually aligned, straight, and not very continuous. Fragments of olivine, siderite, and Mn-siderite are usual, the latter replacing the fossil structures forming the framework (Figure 6 C-6D ). Throughout a transitional contact, the BM II facies overlies the BM I facies which is characterized by their pale green / dark green bluish color and a finer grain size or clayish appearance.
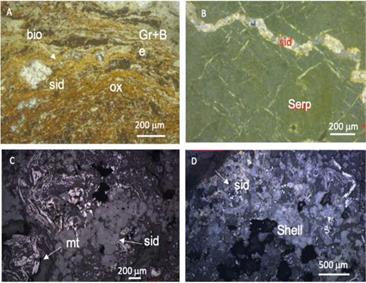
Figure 6 Thin section. A. Red clayish intraclast in which bioconstructions formed of finely stratified filaments composed of oxides replacing old biogenic structures in green matrix of greenalite + berthierine with siderite intraclast can be recognized. B. Serpentine intraclast composed of serpentinite and siderite infilling cracks. C. Black mudstone facies composed of siderite and magnetite. D. Bioclast replaced of siderite into the black mudstone facies.
The framework is composed of bioclast and intraclasts in a similar percentage. According to XRD, BM II facies is composed of magnetite (14%) greenalite (10 to 40%), siderite (30-70%), and chromite (<3%), while the BM I facies I is composed of berthierine + greenalite (55%), siderite (38%), and goethite (7%) (Table 2). Laterally, the black mudstone facies are adjacent and/or overlying the green claystone unit but also weathered peridotites (i.e., green saprolites) and listvenites (i.e., metasomatized peridotites) (Figure 5 C ). Locally, a non-continue succession of red claystone RC composed mainly of goethite with minor fossil content is covering the black mudstone.
EPMA/SEM
A framework with altered fragments of intraclast (e.g., olivine) and bioclast (gastropods) in a clayish matrix (greenalite + berthierine) with abundant Fe-oxides constitute the BM II facies (Figure 7 A-7B ). Shell fossils in a great alteration state are formed principally by siderite. Fe-rich oxides are in fossil structures. According to EPMA results (wd/ed), the siderite replacing shells is composed of Mn and Mg (Mean ± SD, 1.3%±1.0 and 2.5%±1.2, respectively). The associated oxides are goethite and magnetite (white).
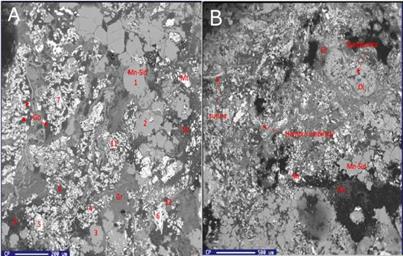
Figure 7 Components of black mudstone and SEM images A. Mn-siderite (Mn-sid), magnetite (Mt), goethite (Go), fragments of olivine (Ol), greenalite (Gr). B. Gastropod fossil, where the umbilical sector and suture are recognized. Additionally, dissolution appearances are observed in olivine.
The mean ± SD FeO content of siderite is 57.7%±4.4 compared to 83.8%±1.7 of magnetite, while the clays present FeO content of 28.6%±7.4. The olivine presents FeO (16.4%±0.4) and MgO (15.4%±4) (Table 3). SEM observations of BM II facies also revealed barite in the clayish matrix (Figure 8).
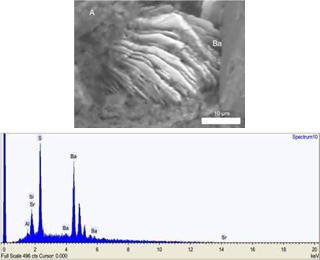
Figure 8 A. Well-formed rhombohedral barite crystals in the fine fraction of the black mudstone facies (SEM image of sample cm- 19 rock ship). B. Spectrum of principal elements forming the barite crystal.
Table 3 wd/ed Chemical composition of principal minerals in black mudstone II facies.
Mineral | Oxides | wd/ed Chemical composition % | |||
---|---|---|---|---|---|
Max | Min | Mean | Desv | ||
K2O | 0.031 | 0 | 0.006 | 0.009 | |
Na2O | 0.301 | 0 | 0.052 | 0.091 | |
Siderit | MnO | 4.078 | 0.175 | 1.309 | 1.063 |
SrO | 0.021 | 0 | 0.002 | 0.006 | |
CaO | 0.174 | 0.02 | 0.069 | 0.044 | |
MgO | 5.183 | 0.958 | 2.505 | 1.217 | |
FeO | 57.594 | 37.622 | 50.362 | 4.492 | |
BaO | 0.04 | 0 | 0.008 | 0.012 | |
K2O | 0.025 | 0 | 0.002 | 0.006 | |
Na2O | 0.113 | 0 | 0.023 | 0.03 | |
MnO | 0.549 | 0.054 | 0.262 | 0.147 | |
Magnetit | SrO | 0.018 | 0 | 0.003 | 0.005 |
CaO | 0.029 | 0 | 0.008 | 0.009 | |
MgO | 0.064 | 0 | 0.022 | 0.025 | |
FeO | 86.159 | 77.841 | 83.88 | 1.712 | |
BaO | 0.065 | 0 | 0.019 | 0.021 | |
K2O | 0.036 | 0 | 0.009 | 0.009 | |
Na2O | 0.916 | 0 | 0.173 | 0.238 | |
Clays | MnO | 1.651 | 0.301 | 0.775 | 0.33 |
SrO | 0.053 | 0 | 0.014 | 0.016 | |
CaO | 0.128 | 0 | 0.032 | 0.028 | |
MgO | 3.984 | 0.891 | 1.683 | 0.795 | |
FeO | 39.461 | 10.789 | 28.617 | 7.423 | |
BaO | 0.078 | 0 | 0.022 | 0.029 | |
K2O | 0.015 | 0 | 0.005 | 0.007 | |
Na2O | 0.051 | 0 | 0.01 | 0.023 | |
Relicts olivines | MnO | 0.156 | 0.126 | 0.144 | 0.011 |
SrO | 0.857 | 0.762 | 0.816 | 0.039 | |
CaO | 0.021 | 0 | 0.011 | 0.008 | |
MgO | 18.493 | 10.435 | 15.446 | 4.035 | |
FeO | 16.767 | 15.908 | 16.444 | 0.394 | |
BaO | 0.066 | 0 | 0.016 | 0.029 |
Stable Isotopes
The bulk sediments isotope results from gf-C facies have δ18O values of −8.7 to −1.8‰ (V-PDB) and δ13C values of -24.0 to −1.1‰ (V-PDB). Siderite concretions in the green fossiliferous claystone have δ18O and δ13C values of −6.4 to −4.1‰ (V-PDB) and −16.0 to −6.0‰ (V-PDB), respectively (Table 4). The dispersion of δ13C vs δ18O data in the graphic corresponds to different rocks type (Figure 9). The meteoric siderite line (MSL) is drawn as a reference. The bulk sediments isotope analyses of the black mudstone unit indicate δ18O values of −14.6 to −1.0‰ (V-PDB) and δ13C values of −21.6 to −8.1‰ (V-PDB), while their intraclast or fragments of green and red claystone have δ18O and δ13C values of -10.2 to -4.3‰ (V-PDB) and −27.1 to -16.0‰ (V-PDB), respectively (Table 4).
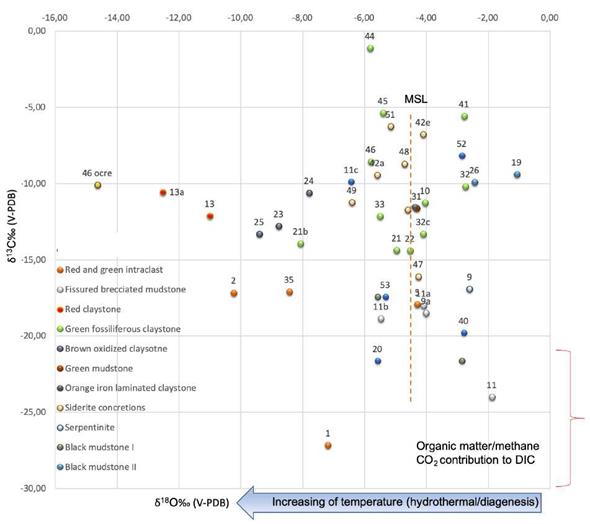
Figure 9 δ13C/δ18O (V-PDB) composition of mound succession formed by brown oxidized claystone, green fossiliferous claystone within siderite concretions, and fissured brecciated mudstones facies and the tabular succession formed by orange iron laminated claystones, black mudstones I and II facies and red claystone. For comparison, Meteoric Siderite Line (MSL) have been plotted (vertical dashed line). For the calculus we have used the equation of Carothers et al. (1988) considering surficial temperatures 25ºC and low latitude water around -4‰ (V-SMOW). Considering the intervention of marine or diagenetic waters more enriched than 0‰, the calculated temperatures are always above the environmental ones.
Table 4 δ13C and δ18O isotope temperature dependent and modeling calculations for the isotope behavior.
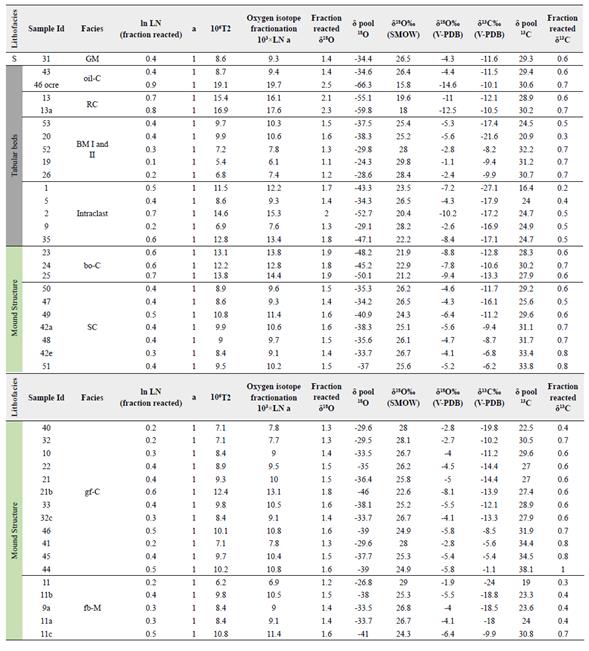
The temperature estimation involved in the sedimentary minerals in Cerro Matoso was determined using the equation of Carothers et al. (1988) . The relationship between the fractionation factor and temperature indicates that, depending on the δ18O values, the green sediment precipitates at temperatures between 25 to 90°C while the black sediments reach 130ºC (Figure 10).
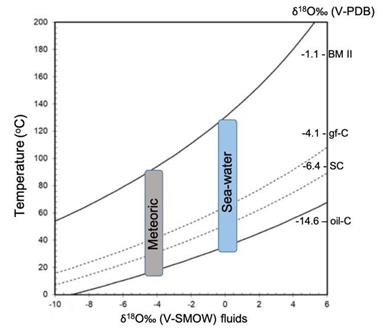
Figure 10 Temperature vs δ18O (water) curves for different δ18O (siderite) compositions, according to the equations of Carothers et al. (1988) . Vertical areas of marine water (0‰ vs V-SMOW) and low latitude meteoric water around -4‰ (V-SMOW) have been plot for comparison. Curves that relate temperature and isotopic composition of water for different values of siderite are represented.
XRF
The black mudstone facies is composed of silica (SiO2 = 2.7-12.4 wt.%), high concentrations of iron (Fe2O3 = 60.4-79.2 wt.%) and low concentrations of lithophile elements such as Al or Ti (Al2O3 = 2.5- 5.3 wt.%). In the red claystone interval, the content of Al2O3 is 15.3 wt.%. Intraclasts forming black conglomerates mudstone facies have silica (SiO2 = 10.8- 23 wt.%) and iron (Fe2O3 = 53.8- 72.3 wt.%) that have similar content to fossiliferous green claystone facies. In the latter, the Al2O3 is less than 4.8 wt.%. These facies have MgO from 2.4 to 5 wt.%. All facies have similar Ba content (Ba = 125.9- 189.6 µg) and variable concentrations of Ni, Cu, Cr, Rb, Sr, and Zn. For comparison, the chemical analyses of mound structure and tabular bed units are in Table 5.
Table 5 XRF Chemical composition and Fe, Ti, Al, and Mn ratios of representative samples of Cerro Matoso sediments.
% | Index (Boström and Peterson, 1969) | |||||||||||||||||||
---|---|---|---|---|---|---|---|---|---|---|---|---|---|---|---|---|---|---|---|---|
Na me | Sam ple Id | SiO2 | Al2O3 | Fe2O3 | MgO | CaO | Na2O | K2O | TiO2 | P2O5 | MnO | Al/ Al+Fe+Mn | 100×Al/ (Al+Fe+Mn) | Al | Fe | Mn | Ti | Mn/Fe | Fe/Al | Fe/Ti |
oil-C | 43* | 2.7 | 3.9 | 60.4 | 0.2 | 0.06 | 0 | 0 | 0 | 0.028 | 0.28 | 0.092 | 9.2 | 2.2 | 21.1 | 0.217 | 0 | 0.01 | 9.8 | 0 |
RC | cm-13 | 9.78 | 15.37 | 70.45 | 1.56 | 0.07 | 0.08 | 0 | 0.63 | 0.18 | 0.02 | 0.257 | 25.7 | 8.5 | 24.6 | 0.015 | 0.378 | 0.001 | 2.9 | 65.2 |
BM II | cm-19 | 8.92 | 5.31 | 79.27 | 1.81 | 0.08 | 0.08 | 0 | 0.05 | 0.01 | 1.02 | 0.094 | 9.4 | 2.9 | 27.7 | 0.79 | 0.03 | 0.028 | 9.4 | 925 |
Serp intr. | cm-20 | 23.05 | 4.82 | 67.31 | 1.75 | 0.13 | 0.08 | 0 | 0.05 | 0 | 1.29 | 0.098 | 9.8 | 2.7 | 23.5 | 0.999 | 0.03 | 0.042 | 8.8 | 785.5 |
CRC intr. | cm-27 | 19.87 | 4.47 | 71.25 | 1.75 | 0.08 | 0.08 | 0 | 0.04 | 0.12 | 0.58 | 0.089 | 8.9 | 2.5 | 24.9 | 0.449 | 0.024 | 0.018 | 10 | 1039.3 |
BM I | cm-25 | 12.42 | 2.52 | 76.17 | 1.63 | 0.17 | 0.08 | 0 | 0.03 | 0 | 5.87 | 0.043 | 4.3 | 1.4 | 26.6 | 4.547 | 0.018 | 0.171 | 19 | 1481.5 |
L | cm-14 | 27.54 | 4.24 | 61.89 | 2.39 | 0.25 | 0.08 | 0 | 0.05 | 0.03 | 0.86 | 0.095 | 9.5 | 2.4 | 21.6 | 0.666 | 0.03 | 0.031 | 9.2 | 722.2 |
GM | cm-9 | 33.88 | 10.63 | 39.13 | 4.03 | 5.67 | 1.27 | 0.08 | 0.47 | 3.15 | 0.16 | 0.3 | 30 | 5.9 | 13.7 | 0.124 | 0.282 | 0.009 | 2.3 | 48.6 |
bo-C | cm-11 | 22.25 | 17.4 | 53.86 | 2.45 | 0.09 | 0.08 | 0.01 | 0.73 | 0.17 | 0 | 0.339 | 33.9 | 9.7 | 18.8 | 0 | 0.438 | 0 | 1.9 | 43 |
gf-C | cm-5 | 10.83 | 8.94 | 72.35 | 4.52 | 0.4 | 0.08 | 0.01 | 0.36 | 0.1 | 0.55 | 0.162 | 16.2 | 5 | 25.3 | 0.426 | 0.216 | 0.017 | 5.1 | 117.3 |
fb-M | cm-1 | 18.39 | 6.71 | 65.81 | 5 | 0.44 | 0.08 | 0 | 0.16 | 0.06 | 0.56 | 0.137 | 13.7 | 3.7 | 23 | 0.434 | 0.096 | 0.019 | 6.2 | 240 |
Chemical composition of the main facies of the metal sediments obtained by x-ray fluorescence in Gmas laboratories. *Data taken from Gleeson et al. (2004) . Black mudstone facies (BM I and BM II), red claystone (RC), orange iron laminated claystone (oil-C), listvenites (L), green intraclast (GC), red cemented intraclast (rc-C).
Trace elements and REE
REE was performed for a sample of black mudstone I and II facies (i.e., sample cm-26 and cm-19 respectively), an intraclast into the BM II facies (i.e., reddish claystone, sample cm-35), oil-C facies that is at the top of the succession (sample cm-43) and for green fossiliferous claystone (gf-C) facies (sample cm- 32). The results are given in Table 6 and Table 7. A Ce* is defined as follows: Ce*= log (Ce/(2/3La + 1/3Nd)) where Ce, La, and Nd represent the shale- normalized values of each element. Ce* ranges from -2.5 to -0.01. The positive Eu anomaly values above 1 are calculated here using Eu/Eu*= 2Eu/(SmN + GdN), where N represents normalization to Post-Archean Australian Shale (PAAS) (Piper, 1974).
Table 6 ICP-MS analyses of tabular bed and mound structure sediments at Cerro Matoso (samples cm-19 and cm-26, cm-32, cm-35 and cm-43).
Analysis method | Detection limit | Analyzed element | Unit symbol | cm-19 BM II | cm-26 BM I | cm-32 gf-C | cm-35 Intra rc-C | cm-43 oil-C |
---|---|---|---|---|---|---|---|---|
TD-MS | 0.01 | Ca | % | 0.03 | 0.06 | 0.15 | 0.07 | 0.02 |
INAA | 0.01 | Fe | 46.1 | 38.4 | 36.8 | 41.9 | 36.5 | |
TD-MS | 0.01 | Mg | 0.66 | 0.72 | 2.05 | 0.53 | 0.25 | |
INAA | 0.01 | Na | < 0.01 | 0.01 | 0.01 | 0.01 | 0.01 | |
TD-MS | 0.01 | K | < 0.01 | < 0.01 | < 0.01 | < 0.01 | < 0.01 | |
INAA | 2 | Au | ppb | < 2 | < 2 | < 2 | < 2 | < 2 |
TD-MS | 10 | Hg | < 10 | 130 | 10 | < 10 | < 10 | |
MULT INAA/TD-ICP-MS | 0.05 | Ag | ppm | < 0.05 | < 0.05 | 0.07 | < 0.05 | < 0.05 |
TD-MS | 0.2 | Cu | 129 | 246 | 68.8 | 75.9 | 60.2 | |
TD-MS | 0.1 | Cd | 1 | 1.3 | 0.2 | < 0.1 | < 0.1 | |
TD-MS | 1 | Mn | > 10000 | > 10000 | 2870 | 5650 | 1590 | |
TD-MS | 0.5 | Pb | < 0.5 | 0.5 | 4.5 | < 0.5 | 1.8 | |
MULT INAA/TD-ICP-MS | 0.5 | Ni | 12500 | 14400 | 7170 | 11500 | 3160 | |
MULT INAA/TD-ICP-MS | 0.5 | Zn | 353 | 407 | 253 | 305 | 157 | |
INAA | 0.5 | As | < 0.5 | < 0.5 | < 0.5 | < 0.5 | 12.6 | |
MULT INAA/TD-ICP-MS | 1 | Ba | 7 | 12 | 13 | 14 | 3 | |
TD-MS | 0.1 | Be | 0.8 | 2.3 | 0.7 | 0.6 | 0.3 | |
TD-MS | 0.02 | Bi | < 0.02 | 0.03 | 0.22 | < 0.02 | 0.49 | |
INAA | 0.5 | Br | < 0.5 | < 0.5 | < 0.5 | < 0.5 | < 0.5 | |
MULT INAA/TD-ICP-MS | 0.1 | Co | 2600 | 3040 | 282 | 385 | 37.2 | |
INAA | 2 | Cr | 9670 | 17500 | 15300 | 23500 | 13800 | |
MULT INAA/TD-ICP-MS | 0.05 | Cs | < 0.05 | < 0.05 | < 0.05 | < 0.05 | < 0.05 | |
INAA | 1 | Hf | < 1 | < 1 | < 1 | < 1 | 2 | |
TD-MS | 0.1 | Ga | 4.2 | 4.8 | 13.2 | 3.9 | 23.8 | |
TD-MS | 0.1 | Ge | 1 | 0.6 | 0.4 | 0.4 | 0.3 | |
TD-MS | 0.1 | In | < 0.1 | < 0.1 | < 0.1 | < 0.1 | 0.2 | |
TD-MS | 0.5 | Li | 0.9 | 1 | 10.9 | < 0.5 | 10.7 | |
TD-MS | 0.1 | Nb | < 0.1 | < 0.1 | 2.6 | < 0.1 | 4.6 | |
TD-MS | 0.05 | Mo | 1.13 | 1.44 | 1.14 | 0.79 | 3.97 | |
TD-MS | 0.2 | Rb | < 0.2 | < 0.2 | < 0.2 | 0.3 | < 0.2 | |
TD-MS | 0.001 | Re | < 0.001 | < 0.001 | < 0.001 | < 0.001 | < 0.001 | |
INAA | 0.1 | Sb | < 0.1 | < 0.1 | < 0.1 | < 0.1 | 1 | |
INAA | 0.1 | Sc | 39.3 | 55.7 | 48 | 46.1 | 58.3 | |
MULT INAA/TD-ICP-MS | 0.1 | Se | 0.2 | 0.6 | 2.4 | 2.4 | 2.7 | |
TD-MS | 1 | Sn | < 1 | < 1 | < 1 | < 1 | 2 | |
TD-MS | 0.2 | Sr | 1 | 2.4 | 3.1 | 2.7 | 1.9 | |
MULT INAA/TD-ICP-MS | 0.1 | Ta | < 0.1 | < 0.1 | 0.2 | < 0.1 | 0.4 | |
TD-MS | 0.1 | Te | 0.2 | 0.3 | 0.3 | < 0.1 | 0.7 | |
MULT INAA/TD-ICP-MS | 0.1 | Th | < 0.1 | < 0.1 | 2.2 | < 0.1 | 4.3 | |
TD-MS | 0.05 | Tl | 0.05 | < 0.05 | < 0.05 | < 0.05 | < 0.05 | |
MULT INAA/TD-ICP-MS | 0.1 | U | < 0.1 | < 0.1 | 0.7 | < 0.1 | 1.7 | |
TD-MS | 1 | V | 89 | 716 | 82 | 25 | 160 | |
TD-MS | 1 | Zr | 2 | 3 | 48 | 2 | 77 | |
INAA | 1 | W | < 1 | < 1 | < 1 | < 1 | < 1 | |
TD-MS | 0.1 | Y | 10.7 | 17.7 | 4.6 | 3 | 1.7 | |
TD-MS | 0.1 | La | 11.5 | 10.3 | 3.9 | 4 | 3.1 | |
TD-MS | 0.1 | Ce | 0.1 | 0.1 | 8.6 | 35.2 | 5.2 | |
TD-MS | 0.1 | Pr | 5.6 | 8.3 | 1.2 | 1.1 | 0.8 | |
TD-MS | 0.1 | Nd | 24.4 | 35.5 | 5 | 4.7 | 2.9 | |
TD-MS | 0.1 | Sm | 6.3 | 9.9 | 1.2 | 1.2 | 0.6 | |
TD-MS | 0.05 | Eu | 1.7 | 3.02 | 0.29 | 0.33 | 0.1 | |
TD-MS | 0.1 | Gd | 4.4 | 8 | 1.1 | 0.9 | 0.4 | |
TD-MS | 0.1 | Tb | 0.7 | 1.6 | 0.1 | 0.1 | < 0.1 | |
TD-MS | 0.1 | Dy | 4.5 | 10.7 | 1 | 0.9 | 0.3 | |
TD-MS | 0.1 | Ho | 0.8 | 2 | 0.2 | 0.2 | < 0.1 | |
TD-MS | 0.1 | Er | 2.4 | 6.5 | 0.7 | 0.5 | 0.3 | |
TD-MS | 0.1 | Tm | 0.4 | 1.1 | 0.1 | < 0.1 | < 0.1 | |
TD-MS | 0.1 | Yb | 2.7 | 8.3 | 0.8 | 0.9 | 0.4 | |
TD-MS | 0.1 | Lu | 0.4 | 1.2 | 0.1 | 0.1 | < 0.1 | |
INAA | 0 | Mass | g | 1.01 | 0.745 | 0.981 | 0.583 | 0.91 |
Note: W, U, Th, Ta, Sb, Sn, Re, Rb, Nb, In, Hf, Cs, Br, As are under the detection limit.
Table 7 Equation form elements normalization of Cerro Matoso (samples cm-19 and cm-26, cm-32, cm-35 and cm-43).
Equations of normalization | cm-19 BM II | cm-26 BM I | cm-32 gf-C | cm-35 Intra rc-C | cm-43 oil-C |
---|---|---|---|---|---|
Ce anom= log (Ce/(2/3La + 1/3Nd)) | -2.52225 | -2.59931 | -0.01508 | 0.60106 | -0.083 |
Eu anom= 2Eu/(Sm + Gd) normalizad PAAS? | 1.37764 | 1.45418 | 1.08109 | 1.35861 | 0.86874 |
(LaSN/YbSN) | 0.36671 | 0.10684 | 0.41973 | 0.38266 | 0.66726 |
LaSN/SmSN | 0.33391 | 0.19032 | 0.59451 | 0.60976 | 0.94512 |
>1 en MORB-E, LaSN/PrSN | 0.50588 | 0.3057 | 0.80061 | 0.89579 | 0.95457 |
EuSN/GdSN | 1.52386 | 1.4889 | 1.03981 | 1.44617 | 0.98602 |
DySN/HoSN | 1.76863 | 1.25677 | 1.20683 | 1.37329 | - |
Discussion
Previous work has shown that the assemblage of berthierine/greenalite + siderite crystallization can be associated with formation conditionsconsistent with high temperatures, alkaline pH, and reducing environments that characterize deep-sea hydrothermal systems, even the Fe-Mn oxyhydroxides have a clear hydrothermal origin (e.g.,Dias and Barriga, 2006; Dias et al., 2011). Fissured and brecciated appearances in the sediments accompanied by a network of microcracks, open and common ducts, and high porosity, with vug and mold porosity, have been associated with the exhalation of fluids. The formation of these sedimentary structures is therefore analogous to fluid-induced chimney formation on the modern ocean floor (e.g.,Eickmann et al., 2021). Although the marine sediments of the upper parts of the oceanic crust accreted to the continent are usually not preserved, the black color and mineral content of the tabular succession plus the fossil content in both successions do not fit with humid tropical conditions of oxidation of peridotites that generate red laterites. Therefore, serpentinization-derived fluids involved in the formation of the mound structures, conduit-filling cement, and tabular bed succession can be supported by these novel data.
The element and stable isotope composition of the sedimentary succession have been used to reconstruct the origin and nature of the fluids from which they are formed. In this sense oxygen, and carbon isotope values of the bulk sediments from Cerro Matoso are consistent with those observed in serpentinization settings of ultramafic rocks (e.g.,Lavoie and Chi, 2010; Lartaud et al., 2011; Klein et al., 2015; Eickmann et al., 2021). Although most of the δ18O values of the gf-C facies are in the range of surface temperatures considering meteoric water (Figure 9), which is supported by the relatively negative δ13C values, samples 32 and 41 present δ18O values indicating temperatures close to 80ºC. Samples of siderite concretions SC are in the same range. The isotopic composition of smectites produced by ultramafic weathering (Savin and Epstein, 1970), with δ18O values (20.3 to 24.3‰ SMOW), in the nickel smectites in Murrin Murrin, Western Australia, represent water-rock interactions at low temperatures due to the contact of meteoric fluids during mineral leaching processes in which laterites are formed (Gaudin et al., 2005). This data show consistency with most of the Cerro Matoso isotope data. Although, marine water or diagenetic fluid relatively enriched in 18O (>-3‰ vs V-SMOW) could explain the temperatures above 50 degrees of samples 32 and 41, a maximum temperature of approximately 90°C, considering meteoric waters are reported in low latitudes close to -4‰ (V-SMOW). Nevertheless, the Ni-laterites formed by supergene alterations of peridotites is a relatively recent process at which the environmental temperatures do not exceed 50°C. Additionally, the wider range of δ18O value (15.9 to 29.8‰ V-SMOW), of the tabular succession also could be explained by surficial temperatures or low temperature hydrothermal/diagenetic system, which fit with the negative δ13C values (-21.6 to -8.1‰ V-PDB) that are compatible with meteoric waters. However, samples corresponding to the BM II facies and their intraclasts report lower values of δ18O (25.1 to 29.8‰ SMOW). These higher temperatures are only justified if fluids are enriched in 18O (magmatic, metamorphic, or diagenetic water).
The lower δ13C values (in intraclast, -27‰ V-PDB) could only be explained by these processes or by the oxidation of methane related to these systems. According to Irwin et al. (1977) , marine/diagenetic waters with relatively negative values of Dissolved Inorganic Carbon (DIC) are formed by the biological degradation of organic matter in closed systems. However, there is no obvious source of significant organic material (e.g., carbon-rich shales) in the sedimentary succession hosting the Cerro Matoso peridotites, so there must be another source of isotopically light DIC. We suggest the production of abiogenic methane by serpentinization of peridotite as the light δ13C source. Methane in modern serpentinite- derived fluids in both the deep-sea and ophiolite environments have negative δ13C values (e.g., -18‰ at Elba and -7.7‰ in the Zambales ophiolite; -16.7‰ at Rainbow; -10.3‰ at Logatchev; -11.9‰ at Lost City; Abrajano et al., 1988; Lilley et al., 1993; Charlou et al., 2002; Proskurowski et al., 2008a; Meister et al., 2018; Sciarra et al., 2019; Eickmann et al., 2021). Part of this methane will be oxidized close to the seafloor to produce 13C-depleted DIC, and high pH and Ca concentrations in the serpentinite-derived fluids trigger carbonate and mineral precipitation on mixing with seawater (Palandri and Reed, 2004; Proskurowski et al., 2008b). The fluids generated during the peridotite serpentinización favored the calcareous sedimentary succession formations on top of the crystalline rocks when these rocks were exposed at a mid-oceanic ridge (Figure 11). Calcareous intraclasts (Id 1, 2, 5, and 35) within the BM II facies, with δ13C values between -27.1 and -17.1‰, indicate a low contribution of marine DIC surely associated with the anaerobic oxidation of methane process (AOM). In this sense, the carbon isotopic composition of Thyasira aff. southwardae shells from “Clamstone” site on Rainbow Hill, with signatures of more depleted 13C (δ13C = −7.69±1.60‰) than expected, is interpreted as a contribution of oxidized methane in the pore-water filling the burrows formed by the bivalves (Lartaud et al., 2010). Our C-isotope data are in the range of serpentinite samples of six different regions in the Mid Atlantic Ridge (MAR) yielded δ13C values ranging from -29 to -4‰ (Früh-Green et al., 2004), which sign methane sources. The rare rock fragments characterized by altered ultramafic lithoclasts (serpentinites) and claystone, are in part similar to those described by Dias and Barriga (2006) for Saldanha sediments, a hydrothermal site of the MAR partly supported by serpentinization processes. According to Lartaud et al. (2011), owing to the very low concentration of inorganic carbon in serpentinization fluids, the most feasible origin for this 13C depleted is the oxidation of methane.
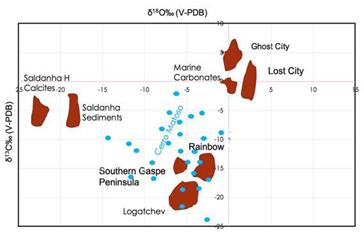
Figure 11 δ13C (V-PDB) vs δ18O (V-PDB) from Cerro Matoso hydrothermal sediments. For comparison, hydrothermal calcite values associated with mineral formation in hydrothermal systems at Lost City (Klein et al., 2015), Saldanha sediments (Dias et al., 2011), carbonates at Ghost City (Lartaud et al., 2011), carbonates and brecciated serpentinites to the south of the Gaspe Peninsula (Lavoie and Chi, 2010); and hydrothermal fluids at Rainbow and Logatchev (Charlou et al., 2002).
Although recently clumped isotope thermometry could constrain the temperature ranges of the carbonates and clays, the carbon isotope data in Cerro Matoso agree in part with the values of δ13C of CH4 and CO2 of high and low-temperature hydrothermal fluids, at 9°50´N East Pacific Rise, with an a verage value of δ13C of -30.2±2.7‰ and -4.5±0.53‰, respectively, which are somewhat more diminished than the average values of δ13C in high-temperature vents, -20.1±1.2‰ of CH4 and -4.08 of CO2 (Proskurowski et al., 2008b). These values are among the lightest measured in hydrothermal vents (Lilley et al., 1993; Shank et al., 1998; Charlou et al., 1998, 2002). In general, carbon dioxide is derived from magmatic degassing, which retains values of δ13C, mainly between -4 and -8‰ (Campbell and Larson, 1998), and carbonates in the mantle, between -5 and -7‰ (Alt et al., 1986; Hoefs and Sywall, 1997), will be related only to the most negative δ13C isotopic signature of the marine sediments in Cerro Matoso because in general, all samples present a wide range of δ13C. The more negative δ13C values in Cerro Matoso samples point out the presence of methane oxidation events, favoring the growth of carbonates in the sediments overlying the ultramafic rocks. Similar conditions have been reported around deep marine hydrothermal vents by Kelley et al. (2001) , Früh-Green et al. (2004) , and Eickmann et al. (2021) .
The initial isotopic carbon ratio of the carbon dioxide discharged from geothermal and ultramafic systems in oceanic ridges, ranges from -8‰ to -3‰, plus the maximum fractionation factor resulting from the oxidation of anaerobic or aerobic methane (1.039) at hydrothermal environments around ultramafic rocks (Whiticar and Faber, 1986; Proskurowski et al., 2008b), could favor a depletion by at least -13‰ of the original isotope signature, giving δ13C values around -21‰ that are consistent with the negative isotopic signature of the minerals forming marine claystone and mudstone in Cerro Matoso. Although the less depleted δ13C values are in the range of meteoric siderite line (MSL) (Figure 8) that could reflect biological processes related to the degradation of organic matter, these values may also record anaerobic oxidation of methane or bacterial oxidation/sulfate-reduction (Irwin et al., 1977). The ancient hydrothermal activity in the Cerro Matoso peridotites is supported by the identification of listvenites (Castrillón and Guerrero, 2020), precisely rocks that are underlying the Fe-rich mudstone succession. If this interpretation is correct, then it suggests that serpentinization-derived fluids flow was active for a considerable time according to the mounds and tabular unit thickness, as well as during the formation of listvenites, and this process was localized in the north area of the Cerro Matoso peridotites.
Geochemical analyses of the sediments show that are associated with high-temperature (i.e., Fe, Cu, Zn, and Ba) but also in elements usually related to hydrothermal low-temperature (Mn), and metasomatism (Mg) in areas where mantle rocks host hydrothermal systems. According to the chemical composition of claystone and mudstone succession, both can be differentiated as hydrothermal or metalliferous sediments. The high iron content of the tabular succession (>80%) plus Cu and Zn permits us to consider it as metalliferous sediments (e.g.,Mills and Elderfield, 1995a; Dekov et al., 2010). Additionally, the criteria of Mn/Fe, Fe/ Al and Fe/Ti ratios used to identify metalliferous sediments (e.g.,Bonatti, 1981; Peter and Goodfellow, 1996; Dekov et al., 2010) indicate that the claystone is in the range of hydrothermal systems and mudstones correspond to metalliferous sediments, respectively. The later, with Mn/Fe > 0.28, Fe/Al > 9.4, and Fe/ Ti > 925 ratios vs Mn/Fe < 0.019, Fe/Al < 6.2, and Fe/Ti < 240 from the claystone facies, also indicate their different origin (Table 5). In general, Dias et al. (2011) suggest that hydrothermal phases derived from low-temperature hydrothermal fluids are characterized by Mn and Fe oxyhydroxides and minor amounts of sulphide precipitates, whereas high-temperature fluids favor precipitation of Cu-sulfides at depth, followed by Cu-Zn and Fe sulfides, which are furthermore characteristic of deep-sea hydrothermal systems. The Al/Al + Fe + Mn has been used to identify the contribution of pelagic sediments to deep marine sediments (e.g., Bonatti, 1981; Dekov et al., 2010). The ratio <0.4 of the Cerro Matoso sediments indicates no contribution of sediments from a continent scenario, which is consistent with the idea of a deep- sea hydrothermal origin instead of a supergene one. In this case, the origin of the metals enrichment could be explained by a plume fall-out source located in an ancient hydrothermal system in the mid-ocean Pacific ridge, where the peridotites were exhumed. The hydrothermal contribution against a detrital in marine sediments has also been estimated using Fe/ Ti vs Al/(Al + Fe + Mn) relation (after Boström and Peterson, 1969; Peter and Goodfellow, 1996) in where Dekov et al. (2010) indicate that all hydrothermal sediments must be on a theoretical curve (Figure 12), in which a Fe/Ti ratio decreasing and Al/(Al + Fe + Mn) increasing sign a dilution of metal sediments with pelagic deep-sea sediments.
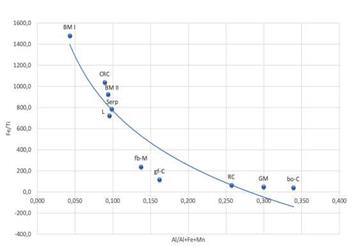
Figure 12 Fe/Ti ratio vs Al/(Al + Fe + Mn) for the sediments of Cerro Matoso which matches the pattern presented by the metalliferous sediments of the Eastern Pacific Dorsa, EPR (adapted from Boström and Peterson, 1969). fb-M: cracked and breached mudstone, gf-C: fossiliferous green claystone, bo-C: brown oxidized claystone, BM I and BM II: black mudstone I and II, RC: red claystone, L: Listvenites, GM: green mudstone, rc-C: red intraclast, Serp: serpentinite intraclast.
A negative Ce (Ce/Ce* = -2.52 to -2.59) anomaly and a positive Eu (Eu/Eu* = 1.37 to 1.45) anomaly that characterize the Cerro Matoso black mudstone sediments have a similar REE pattern of some hydrothermal fluids (e.g., Rainbow’s hydrothermal sediments and transatlantic Geotraversa (TAG), James et al., 1995; Douville et al., 1999, 2002; Dias and Barriga, 2006) (Figure 13). A very high positive Eu anomaly is also marked in many hydrothermal phases (e.g., Fe oxyhydroxides with sulfates and sulphide) derivatives of these fluids (e.g.,Mills et al., 1993; Mills and Elderfield, 1995a; German et al., 1999). On the other side, the pattern of Ce negative anomalies and LREE enrichment characteristic of marine waters (Elderfield, 1988), definitely associate the mudstones with marine environments.
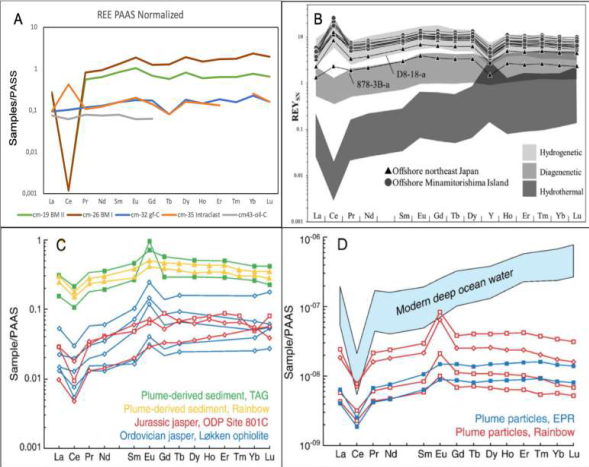
Figure 13 A. PAAS normalized REE patterns of Cerro Matoso sediments: Black mudstone I and II (BM I and BM II), intraclast (rc-C), fossiliferous green claystone (gf-C), orange iron laminated claystone (oil-C). B. Shale-normalized REY spider diagram for the Fe-Mn crusts. Hydrogenetic (Hein et al., 2012), diagenetic (Bau et al., 2014), and hydrothermal (Bau et al., 2014) REY data fields are also plotted. C. Hydrothermal plume-derived iron oxide sediment from the TAG hydrothermal field, Mid-Atlantic Ridge (German et al., 1993) and the Rainbow vent field of the Mid-Atlantic Ridge (Chavagnac et al., 2005), Ordovician jasper from the Løkken district, Norway (Grenne and Slack, 2003, 2005), and Jurassic jasper from ODP Site 801C (Alt et al., 2003) in the western Pacific Ocean (unpublished ICP-MS data of O.J.R.). D. Modern deep ocean water (Byrne et al., 1996), and hydrothermal plume particles from the Rainbow vent field of the Mid-Atlantic Ridge (Edmonds and German, 2004) and the East Pacific Rise at 9°45′N (Sherrell et al., 1999). PAAS data from McLennan (1989) and C, D, modified from Slack et al. (2007).
Hongo et al. (2007) consider that both anomalies indicate a dilution of a hydrothermal fluid signature and removal activity of ocean water particles, reported in mid-ocean ridges. A marked depletion in Ce relative to Pr and La (Shale-normalized patterns) reported here are similar to metalliferous sediments at the EPR, characterized by Bender et al. (1971) with a distinct enrichment in the heavy REE (HREE) relative to the light REE (LREE) (Piper and Graef, 1974; Marchig et al., 1982). Rare earth elements (REE) concentrations are low and PAAS-normalized patterns are characteristic of high-temperature vent fluids with enrichment in light REE and a pronounced positive Eu anomaly (Dias et al., 2011). The REE pattern could be compared with those of iron formations or BIFs (Archean and Early Proterozoic), characterized by high light REE/heavy REE ratios and positive Eu anomalies, which have been interpreted as reflecting hydrothermal sources in mid- oceanic ridges (MOR) (Beukes and Klein, 1990; Bau and Dulski, 1999; Johnson et al., 2003). Barrett and Jarvis (1988) , indicate that the seawater-like pattern of EPR sediments can result from the rapid precipitation and adsorption of seawater REE onto hydrothermally derived Fe-Mn-oxyhydroxide colloidal particles after the particles have settled onto the sea floor or even in the water column. In this sense, Klinkhammer et al. (1983) infer that LREE are removed from the seawater by hydrothermal circulation. The different LREE depletion degrees between samples cm-19 and cm-26 can be interpreted as an effect of the initial reaction that removed LREE from the seawater. These reactions preferentially remove LREE from sea water, leaving the reacted (or scavenged) seawater of the plume even more LREE depleted (Klinkhammer et al., 1983). But also mean a continued post-depositional diagenetic reaction that can involve scavenging from pore water which may increase the proportion of LREE to HREE in the sediment (Barrett and Jarvis, 1988). Additionally, REE/Fe ratios of the BM I (3.3 × 10- 4) and BM II (1.7 × 10-4) facies are quite similar to REE/ Fe ratios of Rainbow hydrothermal field particles (REE/Fe = 1.6 × 10-4) (Chavagnac et al., 2005).
Considerable variation in REE concentration in the black mudstone facies could indicate the existence of a hydrothermal plume that contained many suspended metals, which sequestered rare earth elements (REE) from ocean water and then precipitated within the mudstone. On the other side, the high carbonate concentration in the samples cm-19 and cm-26 suggests that fluid REE fractionation could be induced by coprecipitation with hydrothermally originated minerals (e.g., carbonate), and later by adhesive removal by Fe and/or Mn oxide particles. At any case, REE corroborates that metal sediments inherit their REE signature from deep-sea waters where they precipitate (Dubinin, 2004), and are evidence that claystone and mudstone at Cerro Matoso Pit-1 were formed in a deep-marine setting.
A positive Ce anomaly of Ce of intraclasts (rc-C) (i.e., sample cm-35), corresponds to an anomaly related to a hydrogenic origin (Azami et al., 2018) or according to Tostevin et al. (2016) , could represent deep-sea Fe-Mn crust. Positive Ce anomaly characterizing the intraclasts into BM II facies indicates that the marine sediments could have slumped during the hydrothermal activity and could be the oldest rocks in the deposits, which is consistent with their relationship in the field. Indeed, except for Ce anomalies, the patterns are similar to those of modern plume-related iron oxyhydroxide particles and sediments as well as Phanerozoic hydrothermal sediments (Slack et al., 2007). Slack et al. (2007), compare the range of anomalies with those of oxide particles in the buoyant (near-vent) and non-buoyant (more distal) parts of modern plumes that are consistent with a model of increasing dilution of hydrothermal fluids by ambient seawater away from vents, with dilution factors on the order of 104 in non-buoyant plume (e.g.,German et al., 1993; German and Von Damm, 2003). McMurtry and Burnett (1975) suggest that this kind of sediments unusually enriched with iron, manganese, and other metals, are correlated with areas of high heat flow on oceanic ridges where circulate hydrothermal fluids are generated during mid-oceanic rift volcanism.
The geochemical analysis suggests that a deep- sea hydrothermal activity hosted by the ultramafic rocks favored the mantle rocks serpentinization and contributed to the formations of a thick sedimentary succession associated with biological process and the plume vent fall-out depending on the Eh/pH water changes (Figure 14). The peridotites and marine mudstone/claystone were attached to the continent where the full set of rocks suffered the supergene leaching process allowing the nickel laterite formation as we know today. These explain the differences between Cerro Matoso deposit with other nickel laterites in the Caribbean.
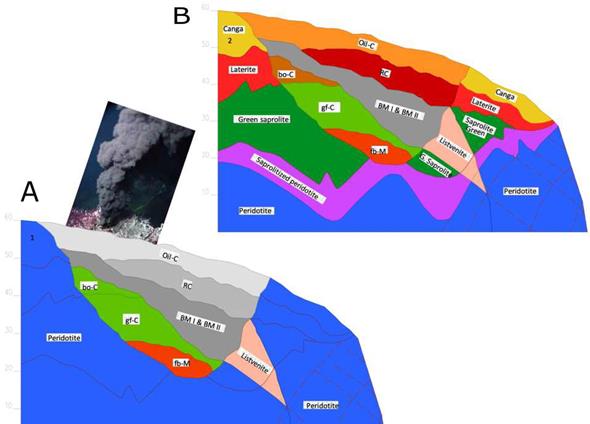
Figure 14 A. Deep-sea ultramafic rock hosting a hydrothermal system where mudstone and claystone were formed. B. Cerro Matoso laterite profile after intense supergene leaching. fb-M: cracked and breached mudstone, gf-C: fossiliferous green claystone, bo-C: brown oxidized claystone, BM I and BM II: black mudstone I and II, RC: red claystone, oil-C: orange iron laminated claystone, L: Listvenites.
Conclusions
The Cerro Matoso sediments, can be understood as ancient examples of a carbonate mineralized seafloor feature that are formed from serpentinization-related seepage in deep-marine settings. δ18O values and temperature-dependent relations (i.e., reaching 130ºC) that present the black mudstone succession indicate precipitation related to a low-temperature hydrothermal fluids influence. The REE patterns, Eu positive and Ce negative anomaly suggest precipitation of a hydrothermal plume fall-out as a source of the black mudstone facies and their hydrothermal phases (oxyhydroxides and silicates) which fit with a serpentinization-derived fluid affecting the mantle rocks of Cerro Matoso. The negative δ13C values confirm deep marine environments in which isotopically light carbon is released from methane and organic matter microbial entry. The very negative CO2 generated contributes to lower the residual isotopic δ13C values of marine waters. The Fe/Ti vs Al/(Al + Fe + Mn) ratios and maximum Al/(Al + Fe + Mn) ratio (<0.4) point out that the Cerro Matoso mantle rocks proceed from the Pacific mid-ocean ridge, where hosted hydrothermal vent plumes responsible for the enrichment of associated metals.