1. Introduction
Alkenes or olefins are traditionally obtained in oil refineries, either from natural gas through extraction processes, or by fluidized bed naphtha catalytic steam cracking. However, due to the constant growth in consumption of olefins and derivatives in the world market, these methods are insufficient. Thus, different synthesis paths to light olefins (C2-C4) production have been studied [1]. The dehydrogenation process offers the possibility to obtain olefins, and it requires high temperatures (>873 K). This condition can generate problems due to thermal cracking side reactions and fast and continuous coke formation; it can imply a total loss of the catalytic activity due to irreversible deactivation or a regeneration step of the catalyst [2].
To overcome the disadvantages of the alkane dehydrogenation process, several alternatives have been proposed, such as the development of catalysts with a higher selectivity and resistance to deactivation or coupling the dehydrogenation reaction (endothermal) with methane oxidation (highly exothermal), to provide the heat required for the dehydrogenation and shift the balance towards alkenes [3]. Nevertheless, the alternative that has aroused more interest is the oxidative dehydrogenation, using oxygen in the reaction mixture to generate olefins, without side or consecutive reactions. In the oxidative dehydrogenation, thermodynamic limitations are overcome, allowing milder operating conditions and avoiding the need for continuous catalyst regeneration. However, the main difficulty is to avoid the consecutive total oxidation to carbon oxides from the light alkane, implying the need for the development of materials with high selectivity [4].
Transition metal oxides are recognized as the best catalysts for oxidative dehydrogenation. Molybdenum, vanadium and cobalt oxides show catalytic activity from 473 K [5]. Vanadium pentoxide is the most studied system. It does not present catalytic activity as a bulk material; the selectivity to propene obtained with supported V2O5 systems is between 60 and 80% and conversions about 1-15% [6-9]. The performance of niobium compounds is similar to that observed with vanadium compounds [10, 11].
Molybdenum compounds have shown low catalytic activity; nevertheless, different molybdates, such as NiMoO4, have higher conversions than those obtained with vanadium-based materials, with a lower selectivity [12-14]. Additionally, transition metal mixed oxides of Mo, V, Fe, Co, Ce, Mn, Te have been studied before, and they have shown a good catalytic activity in oxidative dehydrogenation [15-17].
Tungsten-based materials have not been extensively studied in this particular reaction. Mo-W and V-W mixed oxides supported on alumina or silica have been studied providing good selectivity results but not good conversion [18, 19]. Different catalytic systems for oxidative dehydrogenation of propane based on Co and Ni molybdates or tungstates have been tested; these systems showed a good selectivity and a promising propene yield [20, 21].
In this work, bulk materials in Co-Mo and Co-W systems have been synthesized, which are promising as catalytic precursors in oxidative dehydrogenation of propane. The conditions for a good catalytic performance were found, taking into account parameters such as selectivity, conversion, and stability.
2. Experimental
2.1Catalysts preparation
Bimetallic precursors were prepared using a co-precipitation method and were denominated CoMoϕy and CoWsϕy. The synthesis was carried out by preparing solutions of the metal salts, which were mixed and homogenized during the required time to form the gel. The salts used were Co(NO3)2*6H2O (Merk), (NH4)6Mo7O24*4H2O (Merk) and WO3 (Aldrich); tungsten oxide was dissolved with NH4OH, the NH4OH was also used as the precipitating agent. Next, the precipitating agent was added to the mixture, which was allowed to crystallize for 4 h at 353 K. In the end, the solids were recovered by filtration. Table 1 shows the molar gel composition of the precursor materials. The precursors were dried in a convection oven at 373 K. The final catalyst was obtained from calcination of the precursors at the temperatures that were chosen based on the thermal behavior shown below. Calcination temperature was defined at 673 K for CoWsϕy and at 623 K for CoMoϕy.
Hereafter, the precursors or fresh materials are denominated CoMoϕy and CoWsϕy. The catalysts were denominated with the name of the precursor plus the calcination temperature: CoMoϕy623 and CoWsϕy673.
2.2. Materials characterization
X-Ray diffraction was carried out in a Miniflex model Rigaku equipment with Cu source (λ= 1,5418 Å) operated at 40 kV and 30 mA for values between 5 and 40° and with scanning rate 2° min-1. The thermal stability of the materials was studied by thermogravimetric analysis (TGA) and differential thermal analysis (DTA). TGA was carried out in TA Instruments Hi-Res TGA 2920 in a temperature range between 303 K and 1073 K, at a 10 K min-1 heating rate, under inert atmosphere (N2) and DTA was carried-out on a TA Instruments DSC 2920 model using the same conditions as in TGA. Additionally, fourier transform infrared (FTIR) spectra were obtained with a Perkin Elmer Spectrum One equipment. The samples were prepared as wafers containing ~1 wt% sample in KBr. Moreover, the temperature-programmed reduction (TPR) analyses was performed in a Zeton Altamira AMI-70; in this case, the pre-treatment was carried-out by heating the sample (50mg) from room temperature to 573 K under argon atmosphere, and the catalyst reduction took place under a 30 mL min-1 flow of H2 (10 vol %)/Ar mixture, raising the temperature from room temperature to 1273 K at 10 K min-1.
2.3. Catalytic tests
Oxidative dehydrogenation of propane was performed in a quartz reactor, using a propane to oxygen molar ratio of 2:1 in the reactant mixture. Temperatures in the range 473-873 K were used, 0.4 g of catalyst and a space velocity of 50 mL.g-1.min-1 (298 K and 1 atm) were used. For the catalyst that showed the best performance, different space velocities were tested. During each reaction, the effluent of the reactor was analyzed in a Shimadzu GC-9A gas chromatograph equipped with TCD detector and Molecular Sieve 5A and Porapack Q columns. Calculations of conversion, selectivity, and yield of the reaction for each catalyst were carried out. The main products were propene, CO2 and CO, and some traces of CH4 and C2H4.
3. Results and discussion
3.1. Characterization
X-ray diffraction (XRD)
ϕy phase assignation for the material denominated CoMoϕy, Figure 1(a), was performed through comparison between the obtained diffractogram and the one reported by Pezerat [22] and Levin et al. [23]. The diffractogram for CoMoϕy calcined at 623 K corresponds to β-CoMoO4 phase, which has been previously reported by Smith [24] also in agreement with the pattern of the Powder Diffraction File (PDF 21-868) database. According to literature, this phase is stable at high temperatures; therefore, no changes are expected in the structure of the material at temperatures above 623 K. The material denominated CoWsϕy apparently is a semi-crystalline phase or a phase in formation process, Figure 1(b). When this material is calcined, a low crystalline wolframite-type cobalt tungstate (PDF 72-0479) is obtained.
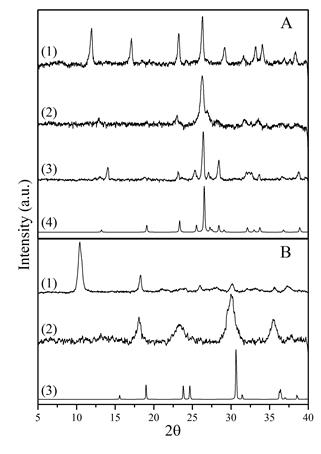
Figure 1 XRD patterns for A: (1) CoMoϕy, (2) CoMoϕy calcined at 623K, (3) CoMoϕy calcined at 873K, (4) β-CoMoO4 pattern. B: (1) CoWsϕy, (2) CoWsϕy calcined at 673K, (3) CoWO4 pattern
Thermal analysis (TA)
For CoMoϕy, Figure 2(a), a weight loss of 11.9% is observed between 373 K and 623 K, which corresponds to two exothermic events related to the evolution of volatile species from the lamellar solid, crystallization water and ammonia, the latter present in the structure as balance cation. In contrast, with CoWsϕy (Figure 2(b)), an apparent single event between 373 K and 673 K is observed, with a weight loss of 5.6%. However, a shoulder is observed at 570 K, the main event at 608 K is assigned to the evolution of crystallization water, and the shoulder is related to a lower amount of ammonium cation present in the CoWsϕy structure. The highest temperatures at which materials no longer present thermal events are 623 K for CoMoϕy and 673 K for CoWsϕy. For this reason, these temperatures were set as calcination temperatures to obtain the final catalysts.
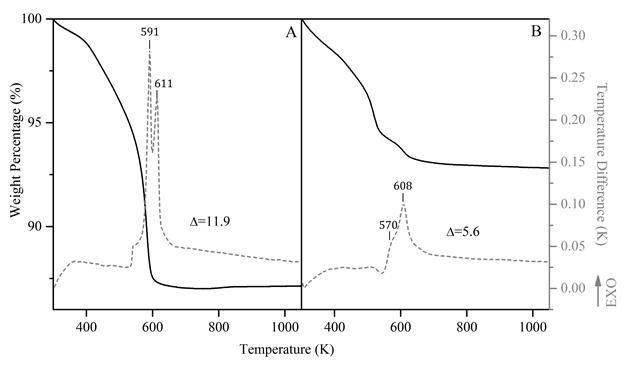
Figure 2 Thermal analysis for (A) CoMoϕy (B) CoWsϕy. Solid lines represent the thermogravimetric analysis (TGA), dash lines represent the differential thermal analysis (DTA)
Temperature programmed reduction (TPR)
The TPR profiles for the calcined materials are presented in Figure 3. For CoMoϕy623 consistency with the reduction temperatures reported by Brito and Barbosa [25] for β-CoMoO4 were observed. It was established that a reduction to lower valence oxidized states takes place, generating equimolar mixtures of Co2Mo3O8 and Co2MoO4 species, followed by a high-temperature reduction to the metals; these events take place at 807 K and 1113 K respectively. Additionally, a shoulder at 973 K is observed, which can be attributed to the reduction of an amorphous phase impurity obtained when calcination of the lamellar precursor took place. This amorphous phase was not observed by X-ray diffraction.
For CoWsϕy673 the reduction events are observed from 673 K upwards, with two peaks at 1073 K, and 1123 K. Complete reduction of the material is achieved at temperatures near 1173 K. This profile is common for tungsten oxide catalysts, which present reduction at temperatures higher than 1073 K [26].
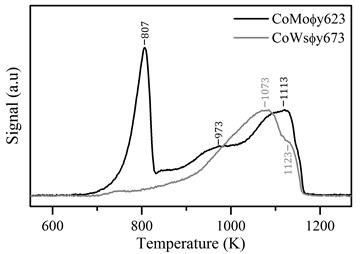
Figure 3 TPR profiles for CoMoϕy623 and CoWsϕy673 by reduction with 10% H2/Ar mixture, from room temperature to 1273 K at 10 K min-1
Fourier transform infrared spectroscopy (FT-IR)
Infrared spectra were obtained for the precursors and the calcined materials (Figure 4). For CoMoϕy, bands at 928 and 620 cm-1 are associated with symmetric O-Mo-O stretching vibrations; the bands at 865, 806, 751 and 475 cm-1 correspond to asymmetric O-Mo-O stretching. All the bands correspond to characteristic vibration modes MoO4 2- tetrahedra. For CoMoϕy623, bands at 943, 841, 784, 704 and 418 cm-1 are observed, which correspond to the vibration bands of β-CoMoO4 structure [27]. This confirms the XRD results, cf. Figure 1(b).
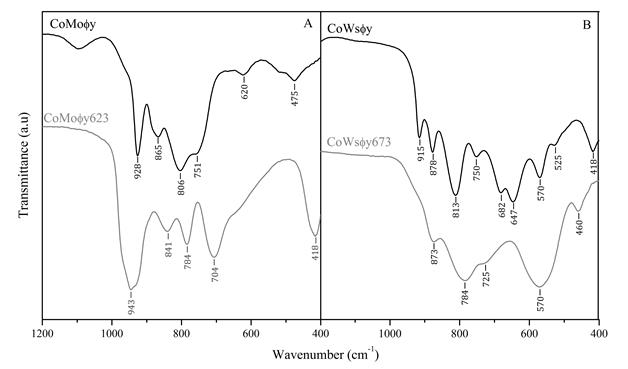
Figure 4 Infrared spectra of the precursors and the calcined materials (A) CoMoϕy and (B) CoWsϕy. Black line precursors, gray line calcined materials
In CoWsϕy spectra, bands at 915, 813 and 418 cm-1 are observed, which agree with the vibration bands observed for scheelite-type oxides, where tungsten is in tetrahedral coordination. Meanwhile, the band at 878 cm-1 is related to symmetric vibration, and the bands at 750, 682, 647, 570 and 525 cm-1 are related to the asymmetric wolframite-type oxide vibrations, where the tungsten has octahedral coordination [28, 29]. For CoWsϕy673, there is a good agreement with the reported information for wolframite [30], indicating octahedral coordination of cobalt and tungsten. The band at 873 cm-1 is related with WO6 6- octahedra symmetrical stretching, while the bands at 784, 725 and 570 cm-1 are related with asymmetric stretching of the same group; the band at 460 cm-1 corresponds to the bending of it. The deformation of the spectra is caused by the low crystallinity of the solid observed in the XRD pattern, Figure 1(b).
Catalytic tests
Figure 5(a) shows that CoMoϕy623 has a measurable catalytic activity from 523 K and CoWsϕy673 from 573 K. The propane conversions are relatively high (Figure 5(a)), taking into account the low temperatures at which catalytic activity was evaluated. The catalyst that presented the best performance, regarding conversion, was CoMoϕy623, with 18.5% conversion at 623 K, while CoWsϕy673 reached conversions smaller than 10% even at 673 K. However, selectivity (Figure 5(b)) at 623 K presents an inverse behavior to that observed with the conversion. Selectivity to propene for CoMoϕy623 tends to be stable at temperatures above 573 K, with a value close to 27%.
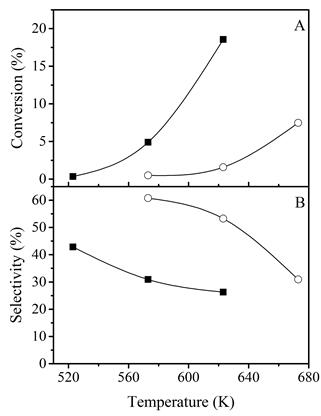
Figure 5 (A) Propane conversion and (B) selectivity to propene as a function of the temperature with a space velocity of 50 mLg-1min-1. CoMoϕy623 (-■-), CoWsϕy673 (-(-)
In order to explore CoMoϕy623 as a catalyst, the reaction temperature was fixed at 623 K, and the space velocity was tested between 50 and 150 mL g-1 min-1. The results are shown in Figure 6. A high space velocity could avoid a total occupancy of the active sites available in the catalyst. Therefore, the conversion could be low. Lower space velocity implies longer contact time; this can increase the conversion and the side reactions. For CoMoϕy, the best yield at 623 K was obtained at a space velocity of 100 mL g-1 min-1. Then, higher temperatures were used to increase the conversion. For this purpose, the precursor material CoMoϕy was calcined at a higher temperature (873 K) and denominated CoMoϕy873. The XRD pattern for CoMoϕy873, Figure 1(a), did not show any significant changes in the structure, and it also corresponds to β-CoMoO4.
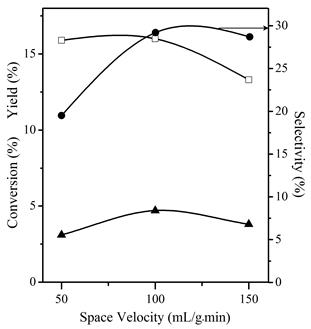
Figure 6 Dependence on conversion (-□-), yield to propene (-(-) and selectivity to propene (-(-) with space velocity for CoMoϕy623 at 623 K
Conversion and selectivity values obtained with CoMoϕy623 and CoMoϕy873, at 100 mLg-1min-1 space velocity to different temperatures, are shown in Table 2. At the same temperature, CoMoϕy873 provided a smaller yield, possibly because of the decrease in the catalyst surface area occurred by the calcination of the precursor at a higher temperature. At higher temperatures, a constant behavior is observed for the selectivity of this catalyst as a function of conversion, within the experimental error. Therefore, the increasing yield obtained with CoMoϕy873 with higher temperature is due to the expected increase in conversion with temperature.
Catalytic stability of CoMoϕy873 was tested at 773 K in continuous reaction for 24 h (see Figure 7] and no loss of catalytic activity was observed. The average conversion value was 20.6%.
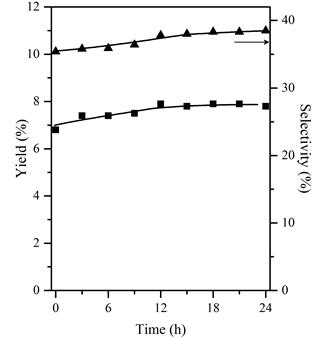
Figure 7 Catalytic stability of CoMoϕy873 at 773 K and a space velocity 100 mL g-1 min-1 yield (-■-) and selectivity (-▲-) to propene. The average conversion value was 20.6 %
The main product of the oxidative dehydrogenation of propane is propene; nevertheless, carbon oxides (CO and CO2) are usually formed as by-products via propane and propene combustion. The propane combustion reaction occurs in parallel with ODH; this explains the inability to reach a 100% selectivity to propene [31]. A consecutive oxidation of propene as a function of the temperature can be explained regarding the bond dissociation energy (BDE). The BDE for the methylene hydrogen is 98 kcal mol-1 in propane and 88 kcal mol-1 in methyl hydrogen in propene [32]; therefore, the transformation of propene to carbon oxides is promoted at higher temperatures. To avoid the oxidation, the propene desorption should be a fast step in the reaction.
The ability of the metal ions to switch between oxidation states, the electronic conductivity of the material, the mobility of lattice oxygen, as well as, the type of oxygen present at the surface are just some of the factors that can affect the activity and selectivity of a catalyst [33]. Even though, a direct comparison of the catalytic activity between CoMoϕy623 and CoWsϕy673 cannot be made for the differences in the composition and structure. The reducibility can be correlated with the redox capacity of materials, which is necessary for the catalytic activity through a Mars-vans Krevelen mechanism. In our case, CoMoϕy623 has a lower onset temperature for the reduction, as well as, the higher catalytic activity in the temperature range studied. The yields achieved with CoMoϕy623 and CoMoϕy873, at different temperatures, are comparable to the ones already reported in the literature; the best yield for cobalt-based materials is around 11% [34-36].
Moreover, the difference in the selectivity, Figure 5(b), value between cobalt molybdate and cobalt tungstate could be attributed to the cobalt, it lies in a high spin state in the tungstate, while it is in a low spin state in the molybdate. This is consistent with cobalt forming an inorganic radical (Co-O*) in the tungstate and not in the molybdate, a species highly active in attacking paraffins, improving the velocity of methylene hydrogen abstraction [37].
4. Conclusions
The synthesis method used is suitable to obtain precursors materials for the catalytic oxidative dehydrogenation of propane. The cobalt- molybdenum precursor material was identified as CoMoϕy. After calcination of the CoMoϕy, the β-CoMoO4 (CoMoϕy623 and CoMoϕy873) phase was obtained. For the cobalt-tungsten precursor material denominated CoWsϕy is a mixture of scheelite-type oxides and wolframite-type oxide according with the FTIR and DRX results. A low crystalline wolframite-type material (CoWsϕy673) is obtained after calcination.
The best catalytic activity, based on selectivity to propene and conversion, was observed for CoMoϕy623.The good catalytic performance of this material was related to a low onset temperature in the hydrogen reduction profiles. This material was tested at higher temperatures, the selectivity was constant, and the conversion increased. This material is promising for the ODH of propane.
The cobalt-tungsten material is interesting because it exhibited a high selectivity, then it is recommended to study other reaction variables to increase the conversion.