1. Introduction
Up to date, analgesics and anti-inflammatories are pharmaceuticals used worldwide to relieve pain and suppress inflammation[1]. Due to the high utilization and continuous excretion through urine, a large fraction of water pollution is associated with anti-inflammatory and analgesic products [2]. They are omnipresent in wastewaters [3]. Acetaminophen (also named paracetamol) is one of the most consumed analgesics and anti-inflammatories. This analgesic is frequently detected in municipal wastewater treatment plants (MWTP) effluents [4,5]. Because of the inability of conventional processes in MWTP to eliminate pharmaceuticals such as acetaminophen (ACE), they end up in the natural water. In the environment, ACE and/or its corresponding metabolites can induce negative influence on aquatic organisms such as fishes, protozoa, and algae even at very low concentrations [2,6,7]. Thereby, technological developments for degrading ACE before its aquatic media input are required to diminish the negative impact of this analgesic on the environment.
Several oxidation processes, including the Fenton system [8], ozonation [9], ultrasound [10], and photocatalytic-oxidation [11], have been proposed for degrading ACE in water. Nevertheless, some inherent disadvantages (e.g., acidic pH requirements, catalysts addition and recovery, metal complexation, high electric energetic demand, or long UV exposure time [8,12,13] limit the application of these methods. A promising alternative is the indirect oxidation by electrogenerated reactive chlorine species (RCS, Cl2,E°: 1.36 V; HOCl, E°: 1.49 V; and OCl−,E°: 0.89 V), which can be used to treat micropollutants and persistent contaminants (such as analgesics) in aqueous matrices [14-21]. The RCS act as oxidizing agents, and they have lower potentials than other oxidants (hydroxyl radical or sulfate radical), which makes RCS more selective for the attack to organic pollutants [14,15,17,22,23]. The use of these species is gaining interest due to they are generated electrochemically from chloride ions present in situ in multiple aqueous matrices (e.g., urine, where chloride ions can be exploited as natural reagents). RCS are mainly produced by the action of dimensionally stable anodes (DSA) such as Ti/RuO2 or Ti/IrO2. These classes of anodes have a large surface area, elevated catalytic activity to the RCS production, low corrosion, and electric energy consumption [24-27].
Previous studies have tested the indirect electrochemical oxidation of ACE [28,29]. However, the application of theoretical analysis of atomic charge (a useful tool to study the reactivity of organic pollutants to the oxidizing species) and ACE degradation in a complex matrix such as urine (the main excretion route for this pharmaceutical) were not reported in these articles. Additionally, the above-mentioned works did not use DSA (which, up to date, are the best anodes for the generation of RCS) in the electrochemical processes. Therefore, this research deals with the elimination of ACE in distilled water and fresh urine by RCS produced on a Ti/IrO2 anode (DSA). Firstly, the ability of the electrochemical system to generate RCS, under modifications of electrolyte-type and current intensity, was tested. Secondly, ACE elimination by indirect electrochemical oxidation was evaluated at favorable conditions of current and supporting electrolyte. Then, the regions on ACE susceptible to attacks of RCS were established through theoretical analyses of atomic charge, and the formation of the primary degradation products is discussed. Finally, the feasibility of the process, to degrade ACE in synthetic fresh urine was considered.
2. Experimental Section
2.1 Reagents
Acetonitrile (HPLC grade), ammonium chloride (99.0%), ammonium heptamolybdate (99.9%), potassium chloride (99.5%), sodium acetate (99.0%), sodium chloride (99.0%), sodium hydroxide (99.0%), sodium sulfate (99.0%), and sulfuric acid (96%) were purchased from Merck. Urea (99.5%) was supplied by M&B Laboratory Chemicals. Disodium hydrogen phosphate heptahydrate (98%), magnesium chloride hexahydrate (99.0%), calcium chloride dihydrate (98.0%), and potassium iodide (99.5%) were provided by PanReac. Formic acid (98.0%) was obtained from Carlo-Erba. Acetaminophen (99.0%) was provided by Laproff laboratories (Colombia). Synthetic fresh urine was prepared according to Amstutz et al. 2012 [30]. [Table 1].
2.2 Reaction system
Degradation of ACE was performed in an undivided cell, containing a Ti/IrO2 (8 cm2 of area) anode and a zirconium spiral (10 cm in length) cathode. Samples of 120 mL were used in all cases, under constant stirring speed (150 rpm) using a magnetic stirrer. The experiments were carried out at least by duplicate. The samples to be treated contained 40 μmol L-1 of ACE (6.05 mg L-1); this concentration was selected taking into account that the analgesics are mainly excreted through urine at mg L-1 levels [31].
2.3 Analyses
The degradation of ACE was determined utilizing a UHPLC Thermo Scientific Dionex UltiMate 3000 instrument, equipped with an Acclaim™ 120 RP-C18 column (5 µm, 4.6 × 150 mm) and a diode array detector (at 243 nm). A mixture of acetonitrile: formic acid (10 mmol L-1) at 15:85 v/v under a flow rate of 0.45 mL min-1 was the mobile phase, and 20 μL of the sample were injected into the UHPLC apparatus.
The RCS accumulation during the treatments was quantified by the iodometry method as reported by Yañez-Rios et al. 2020 [32]. In this method, 600 μL of the sample reacted with 1350 μL of 0.1 mol L-1 potassium iodide, and 50 μL of 0.01 mol L-1 ammonium heptamolybdate. After 5 min of reaction, the absorbance at 350 nm was established using a UV5 Mettler-Toledo spectrophotometer.
Cyclic voltammetry tests were performed using an Autolab PGSTAT 30 apparatus, in a conventional three-electrode cell. The Ti/IrO2 anode was used as a working electrode, Ag/AgCl (3.0 M KCI) as the reference electrode, and the zirconium spiral as the counter electrode. The measurements were conducted at a sweep rate of 50 mV s-1.
The theoretical analyses of atomic charge for ACE were performed using a free online atomic charge calculator. Empirical atomic charges that respond to changes in molecular conformation are calculated via an efficient implementation of the well-established electronegativity equalization method (EEM). The atomic charges in ACE (coordinates from wwPDB CCD entry TYL). The total molecular charge of zero was used. The EEM parameter set Ouy2009_elem covered the NPA charge definition at the B3LYP/6-31G* level of theory[33].
3. Results and discussion
3.1 Effect of applied current density and electrolyte type on the electrochemical generation of reactive chlorine species
Chloride and sulfate are very common ions in aqueous matrices such as urine [30]. Moreover, these anions are frequently used as supporting electrolytes in several processes [17,34]. Hence, at first, the effect of chlorine and sulfate ions on our electrochemical system was tested. Electrolysis experiments for distilled water containing sodium chloride or sodium sulfate (at 0.05 mol L-1, pH: 6.0, and i: 40 mA, conditions based on previous work [35] were carried out, and the accumulation of electrogenerated oxidants was measured [Figure 1A). When the electrolysis was performed with sodium chloride, a significant accumulation of oxidants (RCS) was found (166 and 324 µmol L-1 at 5 and 10 min of electrolysis, respectively). On the contrary, for the electrolysis of distilled water in the presence of sulfate, the electrogeneration of oxidants was close to zero.
The high accumulation of oxidizing species was related to the oxidation of chloride toward chlorine (Equations 1-4) on the Ti/IrO2 anode surface, plus the hydrolysis of chlorine molecules to produce hypochlorous acid and hypochlorite anion (Equations 5-6]. On the other hand, the utilized anode cannot oxidize sulfate ions, but this induces oxidation of water, producing the oxygen evolution (as denoted by Equations 7-9] [21]. It should be mentioned that the oxygen evolution on our DSA is diminished in the presence of chloride and favored by the sulfate ion [24-25].
As the performance of the electrochemical systems is also dependent on the electric current input [36], the effect of this parameter on the electrochemical production of the RCS was considered in our work. Thus, distilled water containing 0.05 mol L-1 of NaCl, was electrolyzed at three current intensities (i: 10, 25, and 40 mA, and potentials: 3.70, 4.75, and 5.67, respectively). The current intensity was varied between 10 and 40 mA, to avoid an excess of the parasitic oxygen evolution reaction (OER, Equation (9)] and limit the formation of toxic chlorine species (such as chlorate) and high electric consumption. Figure 1B shows that the concentration of RCS after 10 min of electrolysis increases from 135 to 324 µmol L-1 as i grows up from 10 to 40 mA. The increment in the current intensity favors the movement of the electrons, and consequently, the generation of RCS (Equations 1-4). Nevertheless, an excessive augmentation of i has disadvantages since side processes like OER (Equations 7-9] or other parasitic reactions (as chlorate formation [37,38] may decrease the current efficiency and increase the electric energy consumption.
Based on the above results, we can indicate that for our electrochemical system, NaCl as the supporting electrolyte, and 40 mA of current intensity are suitable settings that favor the electrogeneration of the RCS. Therefore, these conditions were applied to the system for the treatment of the target analgesic.
3.2 Degradation of ACE by the electrochemical system
After evaluation of the electrolyte type and the current intensity effects, the treatment of ACE was assessed. Figure 2A presents the evolution of ACE under the electrochemical oxidation system, in distilled water in the presence of NaCl or Na2SO4 (at 0.05 mol L-1 and 40 mA). The process using NaCl as the supporting electrolyte led to the complete degradation of the target pollutant after 10 min of electrolysis (equivalent to an electric charge of 0.056 Ah L-1). Meanwhile, when Na2SO4 was used, only 10% of ACE was degraded after 10 min of treatment.
In addition to the ACE evolution, the accumulation of oxidizing species in both presence and absence of the pharmaceutical, for the system with NaCl, was determined [Figure 2B). In the ACE presence, the concentration of the oxidant was ~23% lower than in the pharmaceutical absence. This indicates an interaction of the acetaminophen with the electrogenerated RCS [17,34]. Moreover, the treatment of ACE using Na2SO4 as the supporting electrolyte (the system where RCS are not produced) indicates that the contribution of the direct degradation route through electrons transfer from the pollutant to the anode is low at short treatment times such as 10 min.
To better clarify these aspects, cyclic voltammetry analyses were performed [Figure 2C). Cyclic voltammetry tests using the Na2SO4 electrolyte (red line) exhibit a small wave at ~0.6 V, which can be related to the oxidation of ACE on the anode surface, and the predominant peak at ~1.5 V is the OER. On the other hand, when NaCl was used as an electrolyte (blue line), a wave around 0.7 V was recorded (this can also be associated with the direct oxidation of ACE on the anode surface). Furthermore, the peak at ~1.5 V corresponds to the mix of OER and chlorine evolution reaction (both water and chloride oxidations occur at very close potentials, and as the water concentration is much higher than Cl-, the OER peak masks the chloride oxidation; thus only a big signal is observed, [24,25].
At the experimental pH (6.0), in the system with NaCl as the supporting electrolyte, HOCl is the predominant reactive chlorine species (Equations 5 and 6). Then, this agent is the main responsible for ACE degradation in the electrochemical system, and at the current intensity used in this work (i.e., 40 mA), the degradation through electron transfers from the pollutant to the anode occurs [Figures 2A and 2C), but its contribution is low.
It is reported that the hypochlorous acid is a powerful oxidizing agent (E°= 1.49 V vs. SHE) able to attack organic pollutants as ACE [23]. From Figure 2B, it can be noted that the half-life time of ACE is ~3.5 min, which is a very short time. This can be associated with the strong degrading action of the electrogenerated HOCl. Pinkston and Sedlak have studied the degradation of acetaminophen by direct chlorination, showing under such a system ACE has a half-life time of 5.2 min [39]. Similarly, Bredner and MacCrehan informed the treatment of ACE by chlorination, founding a half-life time of 7.2 min in pure water, and an effective degradation in wastewater [40]. Also, it is informed in the literature that as the amount of chlorine species in water is increased, the ACE degradation is higher [41]. The comparison of our results with literature evidenced the usefulness of electrogenerated RCS to fastly degrade ACE.
3.3 Regions on ACE attacked by HOCl, and possible primary degradation products
As an initial approach to understanding the action of the electrogenerated RCS on ACE, theoretical calculations were done. Analyses to determine the regions on the pharmaceutical, susceptible to attacks by the RCS, were performed on the free online atomic charge calculator[33], and the results are presented in Table 2. A more negative value for the atomic charge means a higher electron density.
The theoretical analysis of atomic charge for ACE indicated that the amide group has the highest electron density (which suggests such moiety on the pharmaceutical is the most susceptible to attacks by electrophilic species such as HOCl). Besides, oxygen in the hydroxyl and some carbons (C4, C5, C7, and C8) on the aromatic ring could also be attacked by the electrogenerated HOCl. However, the amide group has a charge more negative than the phenyl moiety [Table 2]. The amide group is more susceptible to oxidation than phenyl due to nitrogen is less electronegative than oxygen [42]. Additionally, the results of the atomic charge analysis for ACE were consistent with the reported reactivity of chlorine species such as hypochlorous acid, which attacks preferentially activated amides, phenols, and reduced moieties on the structure of organic contaminants [23].
In addition to the theoretical calculations, the chromatographic detection of some primary stable transformation products was done. Figure 3 contains the chromatogram for ACE after 7 min of electrolysis. It can be noted that the electrochemical process produced three primary degradation intermediates (named 4.9, 5.9, and 9.5). Two products appear at lower retention times (4.9 and 5.9 min, respectively) than ACE (retention time: 6.3 min), while a third intermediate was more retained in the column than the parent pharmaceutical (retention time: 9.5 min). Considering the nature of the chromatographic method (i.e., reverse phase, see Section 2.3), we can indicate that the products having retention times of 4.9 and 5.9 min were more polar than ACE, whereas the product with a retention time of 9.5 min was less polar than acetaminophen.
Previous works about the chlorination of acetaminophen have reported that the degradation of ACE produces compounds such as 1,4-benzoquinone, N-acetyl-p-benzoquinone imine (NAPQI), and intermediaries from chlorination on the aromatic ring [39,40,43]. NAPQI and 1,4-benzoquinone are more hydrophilic than ACE (as indicated by their Log P-values lower than for ACE, see Table 2]; thus, the peaks at lower retention times than the parent pharmaceutical may correspond to these substances. Meanwhile, intermediaries coming from chlorination are less hydrophilic than ACE [Table 3]; then, the peak at 9.5 min in Figure 3 may be a chlorinated product.
It can be remarked that NAPQI and1,4-benzoquinone come from the attack of chlorine species to the amide group on ACE, which was consistent with the highest electron-rich nature of this functional group (as indicated by the atomic charge analysis, Table 2]. NAPQI formation starts in the interaction of the amide on ACE with the electrogenerated HOCl, for which a six members ring transition state may be proposed [Figure 4A). This transition state evolves to N-chloramide and hydroxyl anion [45]. The released hydroxyl anion can react with the acid hydrogen on the phenyl group, promoting the formation of NAPQI, being the chloride ion a leaving group. The imine group on NAPQI undergoes hydrolysis [40,46], releasing 1,4-benzoquinone and acetamide [Figure 4B).
Regarding chlorinated products, we can indicate that the aromatic ring on ACE is activated to interact with the electrogenerated Hoc. Hence, chlorination of ACE through mechanisms of aromatic electrophilic substitution can occur [Figure 4C). The hydroxyl moiety is ortho and para directing for the substitution promoted by HOCl [23]. However, as the amide group already occupies the para position on ACE, the chlorinations occur on the ortho positions (which is according to the negative atomic charges on C5 and C7 of ACE, Table 2]. Indeed, the chlorination in ortho positions has been also reported for the treatment of ACE by the UV/HOCl process [47], supporting the high susceptibility of such position to the chlorination.
NAPQI is a noxious substance, which can induce hepatic necrosis [40]. In turn, 1,4-benzoquinone is a well-known toxic product [48]. Additionally, products of ACE chlorination have potential toxicity per se due to their organochlorinated nature. Hence, the electrolysis time could be extended to promote the further oxidation of these initial compounds, or a post-treatment with UVC light of the resultant solutions may be applied to promote the formation of other powerful oxidizing agents (such as chlorine radical and hydroxyl radical, from the homolytic cleavage of the electrogenerated HOCl [47]). It is remarked the relevance of these kinds of experiments (treatment at long times or combination with UVC light). These should be performed in future studies to verify the elimination of such primary noxious products.
3.4 Degradation of ACE in urine
Urine is the main excretion route of ACE in humans after consumption [31]. Then, the feasibility of the electrochemical process to degrade ACE in simulated fresh urine was evaluated. Figure 5A compares the degradation of ACE in urine and distilled water.
Table 2 Theoretical calculations on atomic charge for ACE (online software)
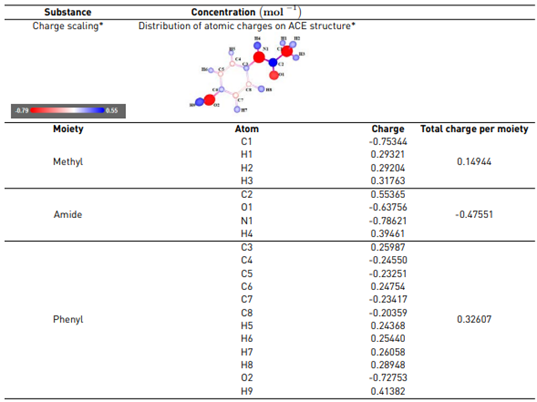
*Information obtained through a free online atomic charge calculator[33].
Table 3 Log P-values for ACE and some degradation products
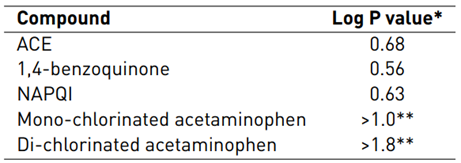
*Log P-value was determined using the free online Molinspiration software[44].
**Data obtained for calculations of chlorine atoms at different positions on the phenolic ring.
As indicated in Table 1, the fresh urine matrix contains a high amount of chloride ions (0.107 mol L-1); thus, it is expected a strong degrading action of the electrogenerated HOCl on ACE. However, the urine also has high concentrations of urea and ammonium/ammonia, and these substances are very reactive toward HOCl (Equations 10 and 11[30,35,49,50]. The reaction of urea and ammonium/ammonia with the RCS is supported by the lower accumulation of electrogenerated HOCl in the urine matrix compared with distilled water (both in the absence of ACE, Figure 5B). In the urine matrix, 21% of ACE was degraded after 60 min of electrolysis. This degradation of ACE is slower than that achieved in distilled water (100% of removal at 10 min of treatment), as a consequence of the competition associated with the matrix components.
4. Conclusions
The treatment of ACE in distilled water by electrogenerated RCS induced a fast elimination (10 min of treatment, under 40 mA of current, 0.05 mol L-1 of NaCl, and using a DSA). The moieties most susceptible to attacks by the RCS on ACE were the amide and aromatic ring. Indeed, the tested treatment could generate 1,4-benzoquinone, NAPQI, and chlorinated acetaminophen as primary degradation products. The process partially degraded the analgesic in urine due to competition between some components in the complex matrix and ACE by the electrogenerated RCS. Therefore, the present work showed that electrogenerated RCS using a Ti/IrO2 anode is an alternative to treat aqueous media loaded with ACE.