Serviços Personalizados
Journal
Artigo
Indicadores
-
Citado por SciELO
-
Acessos
Links relacionados
-
Citado por Google
-
Similares em SciELO
-
Similares em Google
Compartilhar
Agronomía Colombiana
versão impressa ISSN 0120-9965
Agron. colomb. vol.33 no.1 Bogotá jan./abr. 2015
https://doi.org/10.15446/agron.colomb.v33n1.48087
Doi: 10.15446/agron.colomb.v33n1.48087
1 Department of Basic Sciences, Faculty of Natural Sciences and Engineering, Universidad de Bogota Jorge Tadeo Lozano. Bogota (Colombia). carlos.bojaca@utadeo.edu.co
Received for publication: 31 December, 2014. Accepted for publication: 30 March, 2015.
Abstract
Greenhouse tomato production uses structures that protect crops from extreme environmental conditions; however, the climate inside Colombian greenhouses is often not optimal and crops are susceptible to attack by fungal diseases. The use of simulation models for early warnings of attack by diseases have helped to rationalize the use of chemical pesticides by increasing their efficiency when sprayed at critical times of disease onset. The aim of this study was to calibrate the surface wetness energy balance (SWEB) model to estimate the leaf wetness duration (LWD) for greenhouse tomatoes in the Alto Ricaurte province (Boyaca). For the validation, the performances of the SWEB model were evaluated by comparing a simulated LWD with records from dielectric leaf wetness sensors. The model adequately represented the phenomenon of free water on the leaves for plants in two greenhouses of Santa Sofia and Sutamarchan. The model simulated an average LWD of 9.9 and 12.1 hours day-1 in Santa Sofia and Sutamarchan, respectively. However, the simulations for the two greenhouses indicated different behaviors, with average differences between the observed and simulated daily number of hours with free water of 0.8 hours for Santa Sofia, while, for Sutamarchan, the difference reached 4 hours. The fraction of correct estimates index indicated the model had the ability to correctly predict 92 and 72% of the hours with a presence or absence of LWD in Santa Sofia and Sutamarchan, respectively. The SWEB model is a useful tool for early warnings for the attack of fungal diseases in greenhouse tomatoes. However, due to the shortcomings of the greenhouse structures used for production, the crops are highly susceptible to attack from these pathogens.
Key words: epidemiological models, agrometeorology, integrated pest management, early-warning systems, foliar fungal pathogens.
Resumen
Los cultivos de tomate bajo invernadero utilizan estructuras que protegen los cultivos de condiciones ambientales extremas, sin embargo el clima dentro de los invernaderos colombianos, a menudo, no es el óptimo y los cultivos son susceptibles al ataque de enfermedades fungosas. El uso de modelos de simulación que alertan de manera temprana el ataque de enfermedades ha ayudado a racionalizar el uso de plaguicidas químicos aumentando su eficiencia al ser asperjados en los momentos críticos de ataque de la enfermedad. El objetivo del presente trabajo fue calibrar el modelo de balance energético para una superficie húmeda (SWEB) para estimar la duración de la presencia de agua libre sobre el follaje (LWD), en el tomate bajo invernadero de la provincia del Alto Ricaurte (Boyacá). Para su calibración, las simulaciones fueron contrastadas con registros provenientes de sensores dieléctricos de humedad de hoja. El modelo representó adecuadamente el fenómeno de agua libre sobre el follaje en dos invernaderos de Santa Sofía y Sutamarchán. El modelo simuló un promedio de ocurrencia de LWD de 9.9 y 12.1 horas día-1 en los invernaderos de Santa Sofía y Sutamarchán, respectivamente. Las simulaciones para estos dos invernaderos indicaron comportamientos variables, donde la diferencia promedio diaria de número de horas con agua libre sobre el follaje para Santa Sofía fue de 0.8 horas mientras que para Sutamarchán la diferencia alcanzó las 4 horas en promedio. El índice de estimaciones correctas indicó que el modelo tuvo la capacidad de predecir correctamente el 92 y el 72% de las horas con la presencia o ausencia de LWD en Santa Sofía y Sutamarchán, respectivamente. El modelo SWEB es una herramienta útil de alerta temprana para el ataque de enfermedades fungosas en tomate bajo invernadero. Sin embargo, las deficiencias de los invernaderos utilizados para la producción indican un alto grado de susceptibilidad de los cultivos al ataque de estos patógenos.
Palabras clave: modelos epidemiológicos, agrometeorología, manejo integrado de plagas, sistemas de advertencia temprana, patógenos foliares.
Introduction
Early warning disease models are decision-making tools that help growers assess the risk of diseases that can cause economic damage to crops (Gleason et al., 2008). The implementation of predictive disease models is an important integrated pest management strategy to make a rational use of pesticides in agricultural systems (Guzman-Plazola et al., 2003; Gleason et al., 2008).
Infections caused by disease and agrometeorological variables can be related using simulation models, which deliver useful information to improve decision-making about the timing for pesticide applications (Dalla Marta et al., 2005). The main climate variables commonly used in disease prediction models include air temperature, precipitation, relative humidity and the presence of free water on the canopy (Gleason et al., 2008). Leaf wetness duration (LWD) is an important parameter for the successful use of disease prediction models based on climate conditions (Dalla Marta et al., 2005). The LWD term is defined as the time that free water remains on the surface of the foliar tissue (Sentelhas et al., 2006). The presence of free water on the canopy influences biological processes such as water absorption, gas exchange, nutrient leaching, contamination by pollutants, and self-cleaning properties of plants (Aryal and Neuner, 2010).
Microclimatic conditions within a crop largely determine the crop's susceptibility to diseases (Sentelhas et al., 2005). The LWD and relative humidity, promoted by the presence of dew, mist or irrigation, play an important role in many epidemiological events and mainly drive the infection and sporulation processes (Huber and Gillespie, 1992). The presence of a water film on the surface of leaves is a precondition for starting the infection process of several pathogens (Rosa et al., 1995; Orlandini and Rosa, 1997; Rosa and Orlandini, 1997; Sentelhas et al., 2008).
Normally, the presence of dew is the main contributor to LWD. The deposition process on the leaves occurs particularly during the night, when the surface of the leaves has a lower temperature than the dew point of the surrounding air (Sentelhas et al., 2008). The process is the result of heat loss from the surface of the plant to the environment. Radiative heat loss at night is the most important factor involved in the condensation of dew and, for this reason, is an input in many physical models that simulate the occurrence of free water on the canopy and its duration (Luo and Goudriaan, 2000). Although the condensation process may be easily described from the physical viewpoint, the LWD is difficult to measure since it is the result of interactions between the position of the leaves and their arrangement, the canopy structure and the surrounding environment (Sentelhas et al., 2008).
In Colombia, the use of disease prediction models is scarce. The only reference to this subject is the work of Gil et al. (2011), who evaluated four methods for assessing the LWD in greenhouse rose cultivation. They evaluated three empirical models and a physical one based on the work of Pedro (1981), at different heights within the crop canopy. In general, empirical models based on relative humidity and dew point depression thresholds showed better results in terms of accuracy and precision. On the other hand, the simulations with the physical model showed the best results for the LWD observed at 1.2 m. Tomato is one of the most important horticultural crops in Colombia and, for 2013, greenhouse production was carried out on 2,778 ha, with an average yield of 106.4 t ha-1 (Agronet, 2014). Greenhouse production is a relatively intensive system that has adopted practices such as fertigation, regular pruning and the use of training systems (Bojacáet al., 2013). Diseases are controlled with chemical pesticides. The main fungal targets are late blight (Phytophthora infestans) and powdery mildew (Sphaerotheca pannosa) (Bojacá et al., 2012).
Most disease prediction models available in the literature have been developed for open field conditions since, under these conditions, disease pressure and weather conditions are more conducive to the attack of fungal diseases. However, within greenhouses that are used for tomato production in Colombia, the internal climate may become equally suitable for the onset of diseases. Therefore, the objective of the present study was to calibrate the surface wetness energy balance (SWEB) model proposed by Magarey et al. (2006) to quantify the LWD on tomato plants in one of the major greenhouse tomato production regions in Colombia, the Alto Ricaurte province (Boyaca).
Materials and methods
SWEB model description
The surface wetness energy balance (SWEB) model represents the water budget of a crop canopy. The model consists of four sub-modules that describe the: (i) surface water distribution based on an observed wet fraction; (ii) canopy water budget; (iii) energy balance module based on a combination equation developed by Tanner and Fuchs (1968); (iv) a transfer function based on Bird et al. (1960) generic transfer coefficient that was previously calibrated to a surface wetness under controlled conditions.
The surface wetness (SW) is estimated from the comparison between the index of the fraction of canopy wet surface area and a surface wetness threshold for a given interval time. For the present study, the model ran on an hourly basis. The presence of free water on the leaves is represented as SW = 1, while its absence results in SW = 0. LWD is the result of adding up the time periods with SW = 1 during a day. For a full description of the SWEB model, the reader is referred to the original source (Magarey et al., 2006).
As stated by Magarey et al. (2006), the SWEB model can be adapted to any crop by adjusting four parameters: leaf area index (LAI), maximum fraction of canopy allowed as wet surface area (Wmax), crop height and maximum water storage per unit area (C1). In the present study, for the canopies in the two greenhouses, the LAI was set to 3 m2 m-2, Wmax was kept at 0.5 (Magarey et al., 2006), while the crop height, measured in the field, was set to 2 m.
The C1 parameter was determined by spraying water mist on the leaf from a known water volume until it dripped off the leaf. The difference between the initial and final volume in the container corresponded to the maximum volume of water that each leaflet could hold.
The area of each leaflet was determined using the relationship between the number of pixels of a reference surface with a known area and the number of pixels of each leaflet captured in a digital picture. The average C1value of from 30 samples was 4.56 cm, with a range of 1.3 and 9.6 cm.
Additionally, we removed the interception of the precipitation term, which, in our case, was not needed since precipitation was avoided due to the greenhouse cover. Thus, the canopy water budget only depends on the potential dew condensation, since this is the only source of moisture for a greenhouse crop. The model was programmed in the R statistical computing language (v.2.15.2, R Core Team, 2012).
Field data collection
The LWD validation data for the greenhouse tomatoes were collected from two commercial greenhouses in the Alto Ricaurte province. The selected greenhouses were located in the municipalities of Santa Sofia and Sutamarchan (Boyaca, Colombia). Both greenhouses had a traditional ridge and furrow design built with a wooden structure and natural ventilated through fixed openings in the ridge and mobile side windows. The greenhouses were located at altitudes of 2,427 and 2,112 m a.s.l. for Santa Sofia and Sutamarchan, respectively.
The greenhouses were planted with the tomato cultivars Bachue and Ichiban (Seminis Vegetables, St. Louis, MO) in Santa Sofia and Sutarmachan, respectively. Measurements started at the beginning of the harvest phase and extended for a period of 36 (29/08/2012 - 04/10/2012) and 40 (12/12/2012 - 21/01/2013) consecutive days in Sutamarchan and Santa Sofia, respectively. At both locations, the planting density was 2.7 plants/m2 without the farmers applying any fruit-pruning scheme. The transplant was carried out when the plantlets showed an average of 10 nodes and the plants were managed with a single stem by removing all lateral shoots. The crops were managed according to the regular practices applied by the farmer in each greenhouse.
The variables recorded were: leaf temperature, air humidity and solar radiation at heights of 1 and 2 m above the ground, corresponding to the middle and upper thirds of the canopy. For the upper third, these variables were recorded using a compact weather station (Pessl Intruments GmbH, Weiz, Austria). The leaf temperature was recorded with two infrared radiometers (IR, model SI-111, Apogee Instruments, Logan, UT) at each height.
Infrared radiometers were installed at a distance of 5 cm from the target leaf surface, pointing to the center of a leaflet. In the middle third, the air temperature was recorded by means of T-type thermocouples (Copper-Constantan) connected to a data logger (Cox-Tracer Junior, Escort DLS, Edison, NJ). The thermocouples were located within white PVC cylinders to reflect direct sunlight. The relative humidity was calculated through the relationship between the air temperature and the one recorded by a thermocouple inside a wet bulb using a psychrometric chart. Additionally, at this height, a solar radiation sensor was installed. In each greenhouse, we installed a set of dielectric leaf wetness sensors (LWS-L, Decagon Devices, Pullman, WA) at the same heights as the other sensors.
At each height, two LWS-L sensors were installed, recording the integrated output voltage at regular time intervals of 10 min. The threshold voltage recorded by LWS-L sensors, necessary to establish whether the leaves were wet or dry, was determined in the laboratory. Values less than or equal to 271 mV indicated that the leaves were wet (SW = 1), while higher values indicated that the leaf was dry (SW = 0). Dielectric sensors were installed within the canopy of the plants at an incline of 45°, emulating the leaf orientation.
Outside solar radiation and wind speed were recorded with a weather station (WatchDog model 200, Spectrum Technologies, Plainfield, IL). Since the SWEB model requires the wind speed as an input, the wind speed inside the greenhouse (Uz) was estimated based on the outside wind speed (Vout) using the following regression for naturally ventilated greenhouses proposed by Wang et al. (2000):

All inside and outside climate variables were recorded every 10 min and averaged per hour for model simulations.
SWEB model performance
We assessed the performance of the model by comparing the readings of the dielectric sensors versus the model simulations. First, we compared the simulated wet hours versus the results of the dielectric sensors on a daily basis for each greenhouse. To do this, we aggregated the number of wet hours per day for each output source (model or sensors) and applied the exact Wilcoxon signed rank test (Wilcoxon, 1945). Afterwards, we determined the proportion of events classified or misclassified as wet or dry at each locality (Wilks, 1995). These events were used to calculate the indexes: fraction of correct estimates (FC), correct success index (CSI), false alarm rate (FAR) and bias (BS).
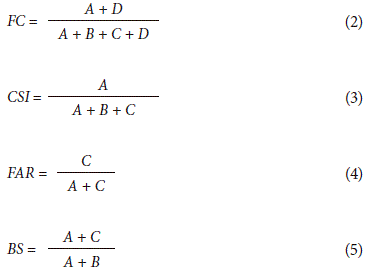
where A is a success (wet event detected by the sensor and correctly predicted by the model), B represents a miss (wet event detected by the sensor but not predicted by the model), C is a false alarm (wet not detected by the sensor but predicted by the model) and D is a correct negative (wet not recorded by the sensor and not predicted by the model).
The first three indexes ranged between 0 and 1. If the model appropriately predicted wet events, the FC and CSI indexes should have values close to 1, while the value of FAR should be close to 0. Finally, the value of BS indicates the bias of the predictions: values greater than 1 indicate an overestimation of the leaf wetness duration.
Results and discussion
Climate conditions
The humidity within the canopy, represented by the relative humidity (Fig. 1A-B) and vapor pressure deficit (Fig. 1C-D), presented a similar behavior between the two greenhouses and heights that were considered. During the daytime, the relative humidity has lower values compared to the night when, due to low temperatures, the air is saturated with moisture reaching full saturation, especially during early morning hours. The average values of relative humidity and vapor pressure deficit at the two measuring heights are presented in Tab. 1. At the greater height, the moisture buildup was higher during most of the day, except during the early morning when the humidity was similar for the two heights.
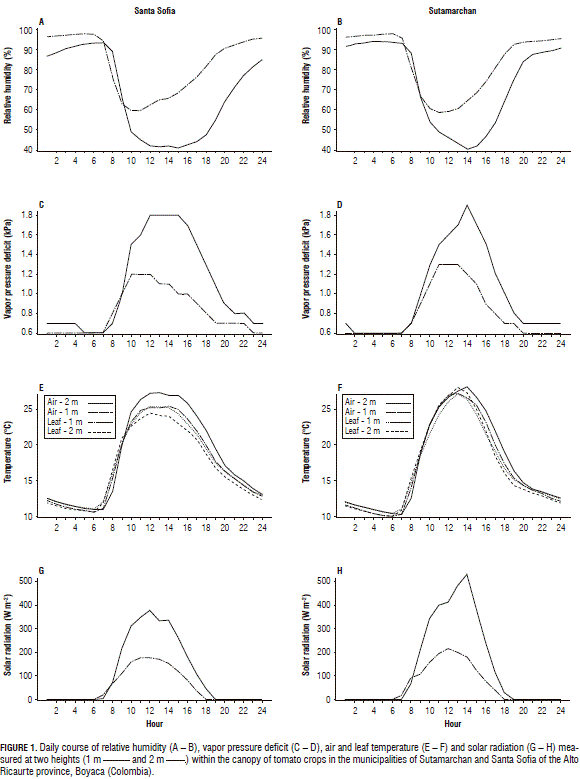
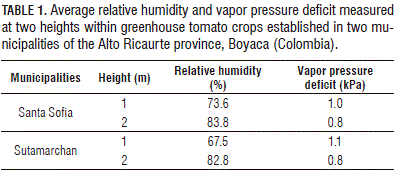
The 24-h trend of the relative humidity within the greenhouses confirms the favorable condition they present for the development of fungal diseases. According to Guzman-Plazola et al. (2003), high levels of relative humidity (80-90%) are favorable for the germination of spores of diseases such as powdery mildew.
In Santa Sofia, the average temperatures were 17.3 and 16.8°C at 2 and 1 m, respectively. Slightly higher temperatures were recorded for Sutamarchan, with averages of 18.3 and 17.4°C at 2 and 1 m, respectively. The temperature differences between the two heights were minimal when the periods of the day and night were compared. In Santa Sofia, the average temperature difference between the two heights for the day (6:00 AM to 5:00 PM) was 0.4°C, while, for the night (6:00 PM - 5:00 AM), it was 0.5°C. Larger temperature differences were observed in Sutamarchan, where the average daytime difference was 1.1°C, while, for the night hours, it was 0.7°C. From the crop growth point of view, previous research has not shown any effect of vertical temperature gradients on the accumulation and distribution of dry matter in greenhouse tomato crops (Qian et al., 2012).
Environmental conditions, similar to those found in the studied greenhouses, such as a high relative humidity coupled with relatively warm days and nights with low temperatures, have been reported as favorable conditions for the occurrence and extended duration of the phenomenon of free water on the canopy and for the development of fungal diseases within greenhouses (Mersha et al., 2014).
In Santa Sofia, there were no differences between the leaf temperatures registered at 1 and 2 m within the canopy. The average leaf temperature was 16.7°C at the two measurement heights. In Sutamarchan, the leaf temperature at 1 m, with an average of 17.5°C, was higher than the one recorded at 2 m where the average was 16.9°C. In Santa Sofia, during day hours, the average temperature at 2 m was 20.9°C, while, at 1 m, it was 20.4°C.
Conversely, in Sutamarchan, the average temperature during the day was greater at 1 m (21.0°C) than at 2 m (20.4°C). During night hours, higher leaf temperatures were recorded at 1 m for the two greenhouses. In Santa Sofia, the average night temperature at 1 m was 12.9°C, while the observed value at 2 m was 12.4°C. In Sutamarchan, the average night temperatures were 14.0 and 13.4°C at 1 and 2 m, respectively.
Fig. 1E-F show how the leaves maintained lower temperatures than the air, during most of the day. At night, the temperature difference between the leaves and the air was reduced. For example, the temperature difference between the air and leaves at 1 m was 0.15 and 0.05°C in Santa Sofia and Sutamarchan, respectively. Recently, it was demonstrated that the leaf temperature has a significant effect on the dynamics of foliar fungal pathogens. Bernard et al. (2013) developed the first temperature curve for a fungal pathogen (Mycosphaerella graminicola on wheat) based on the leaf temperature rather than the air temperature, concluding that the temperature sensed by the pathogen is more representative of the environmental conditions experienced by the pathogen.
The average global solar radiation recorded at 2 m in Santa Sofia and Sutamarchan was 108.4 and 134.0 W m-2, respectively. As a result of the radiation extinction with depth into the canopy, the average global radiation measured at 1 m was lower. In Santa Sofia, the average global radiation at 1 m was 53.4 W m-2, while, in Sutamarchan, it was 59 W m-2. According to these records, the measurement period in Santa Sutamarchan was sunnier, as presented in Fig. 1G-H.
LWD recorded by dielectric sensors
Previously reported climate conditions within the crop favored the occurrence of the phenomenon of free water on the canopy within the two greenhouses. Figure 2 shows the average daily behavior of this variable at two heights in the two greenhouses. The results indicated that, in Santa Sofia, the free water on the canopy occurred during shorter time periods than those observed in Sutamarchan.
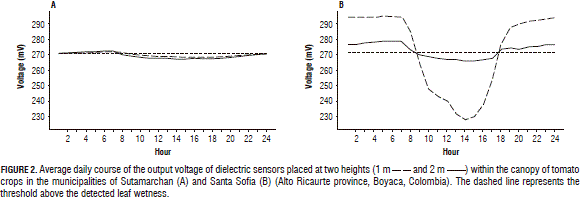
On average, the LWD in Santa Sofia was 9.6 h d-1 according to the dielectric sensors and the predefined threshold of 271 mV. In Sutamarchan, the LWD daily average was 14.4 h for the two heights. As with the previous variables, differences between the greenhouse and heights were due to differences in climate and experimental conditions. The average LWD determined inside a greenhouse growing roses using dielectric sensors was 9.9 h d-1, underestimating the average value of 11 h d-1 determined by direct visual observations (Mashonjowa et al., 2013). The authors attributed this underestimation to factors such as distillation, gutation, dripping of the covering material and residual water irrigation.
As reported by Sentelhas et al. (2005) for coffee and grape crops, the present results indicated no vertical LWD gradient within the canopy. However, the same study found LWD differences regarding the vertical position for apple and corn. Differences in LWD patterns depend not only on the architecture of each species but also on the planting system, crop age and management practices (Sentelhas et al., 2005).
SWEB model performance
The model simulated an average LWD of 9.9 h d-1 in Santa Sofia. The model estimated the occurrence of the phenomenon with variations from 9 to 11 h d-1. The average daily number of hours simulated was very similar to that observed with the dielectric sensors (9.6 h d-1). The model indicated that the LWD phenomenon appeared during the night hours, as shown also by the leaf wetness sensors and as expected according to the climatic behavior of the greenhouse.
In Sutamarchan, the model estimated an average LWD of 12.1 h d-1, registering a greater variation with respect to the results for Santa Sofia. In the greenhouse of Sutamarchan, the LWD ranged between 9 and 16 h d-1 with an incremental behavior, in which, during the first simulated days, the LWD was between 9 and 10 h d-1, while, towards the end of the simulated period, the LWD was between 15 and 16 h d-1. As in Santa Sofia, the model determined the occurrence of LWD during night hours.
The results of the exact Wilcoxon signed rank test indicated statistically significant differences for the daily duration of LWD between the SWEB model and the output of the dielectric sensors. For the greenhouse in Santa Sofia (V statistic = 254.5, p-value = 0.04), the differences between the two methods were closer than the ones observed for the greenhouse in Sutamarchan (V statistic = 146, p-value = 0.002). The average difference of the daily duration of the LWD phenomenon recorded by the sensors and simulated by the model was 0.8 h for the greenhouse in Santa Sofia, while, in Sutamarchan, the difference was 4 h. This comparison confirms the best performance of the model for the climate and greenhouse management practices applied in Santa Sofia.
The SWEB model presented a good degree of fit compared to the readings generated by the dielectric sensors. The trend between the observed and simulated data was similar for most of the measurement period. Table 2 presents the indices constructed to assess the model's accuracy and representation of the phenomenon of LWD in the two greenhouses.
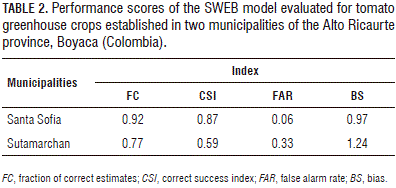
In general, the indices showed a better performance for the SWEB model for the conditions in Santa Sofia. The highest values of both FC and CSI, very close to 1, were indicative of a better performance for the condition in Santa Sofia. The FC index indicated that the model had the ability to correctly predict 92% of the hours in which the phenomenon of LWD occurred, as well as the hours that actually had no free water on the leaves. In Sutamarchan, only 72% of the hours, both with presence or absence of LWD, were correctly simulated.
When considering the proportion of false positive events in relation to all positive (false or true) in Santa Sofia, 87% of the hours that positively occurred in the LWD phenomenon were correctly detected, while, in Sutamarchan, the percentage was only 59%. In contrast, the occurrence of false alarms rate represented by FAR was much lower in Santa Sofía (6%), as compared to 33% in the Sutamarchan greenhouse.
The BS index indicated a small underestimation (3%) of the model in the case of Santa Sofia, while, for the case of Sutamarchan, the model overestimated the hours of free water on the leaves by 24%. This index generally consolidated the results obtained for the other indices and confirmed the better performance of the model for the greenhouse in Santa Sofia.
Conclusions
The present study represents the first attempt to adapt a leaf wetness model to a greenhouse crop. In other latitudes, active climate control strategies, along with a more technically sound crop management, restrict the possibility of fungal attacks. Nevertheless, the environmental conditions that occur in greenhouses used for growing tomatoes in regions such as the Alto Ricaurte province (Boyaca, Colombia) are conducive to the occurrence of the phenomenon of free water on the canopy and, consequently, for the appearance of and attack by fungal diseases. Relatively warm days followed by cool nights and high relative humidity cause condensation of water droplets on leaves, which are particularly deposited during the early morning hours.
The adaptation of the SWEB model to the conditions of greenhouse tomato production in the Alto Ricaurte province was able to adequately represent the phenomenon of free water occurrence on the canopy. The adapted model represents a useful tool for alerting the occurrence of periods with free water on the canopy. Therefore, the availability of an early warning system may contribute to a rationalized use of chemical pesticides to control crop diseases.
However, the occurrence of free water on the canopy during all of the evaluated nights indicated that the attack of fungal diseases throughout the crop cycle was a latent possibility. This risk can only be decreased if the climate conditions inside the greenhouses are dramatically improved. Climate management strategies must include temperature increments during nighttime, as well as the quick removal of water vapor through an optimized ventilation system.
The particular greenhouse climate conditions and the crop management practices of each production cycle generate differences in the intensity of the phenomenon of free water on the leaves. This situation causes the model to under- or overestimate the number of hours this phenomenon occurs during the day.
Acknowledgements
The present study was funded by the Flemish Interuniversity Council (Belgium) through the project ZEIN2009PR364 "Multidisciplinary assessment of efficiency and sustainability of smallholder-based tomato production systems in Colombia, with a roadmap for change".
Literature cited
Agronet. 2014. Estadísticas para el sector agrícola. In: Ministerio de Agricultura y Desarrollo Rural, www.agronet.gov.co; consulted: March, 2015. [ Links ]
Aryal, B. and G. Neuner. 2010. Leaf wettability decreases along an extreme altitudinal gradient. Oecologia 162, 1-9. Doi: 10.1007/s00442-009-1437-3 [ Links ]
Bernard, F., I. Sache, F. Suffert, and M. Chelle. 2013. The development of a foliar fungal pathogen does react to leaf temperature! New Phytol. 198, 232-240. Doi: 10.1111/nph.12134 [ Links ]
Bird, R.B., W.E. Stewart, and E.N. Lightfoot. 1960. Transport phenomena. J. Wiley & Sons, New York, NY. [ Links ]
Bojacá, C.R., H.A. Casilimas, R. Gil, and E. Schrevens. 2012. Extending the input-output energy balance methodology in agriculture through cluster analysis. Energy 47, 465-470. Doi: 10.1016/j.energy.2012.09.051 [ Links ]
Bojacá, C.R., L.A. Arias, D.A. Ahumada, H.A. Casilimas, and E. Schrevens. 2013. Evaluation of pesticide residues in open field and greenhouse tomatoes from Colombia. Food Control 30, 400-403. Doi: 10.1016/j.foodcont.2012.08.015 [ Links ]
Dalla Marta, A., R.D. Magarey, and S. Orlandini. 2005. Modelling leaf wetness duration and downy mildew simulation on grapevine in Italy. Agric. For. Meteorol. 132, 84-95. Doi: 10.1016/j.agrformet.2005.07.003 [ Links ]
Gil, R., C.R. Bojacá, and E. Schrevens. 2011. Suitability evaluation of four methods to estimate leaf wetness duration in a greenhouse rose crop. Acta Hort. 893, 797-804. [ Links ]
Gleason, M.L., K.B. Duttweiler, J.C. Batzer, S.E. Taylor, P.C. Sentelhas, J.E.A.M. Monteiro, and T.J. Gillespie. 2008. Obtaining weather data for input to crop disease-warning systems: leaf wetness duration as a case study. Sci. Agric. 65, 76-87. Doi: 10.1590/S0103-90162008000700013 [ Links ]
Guzman-Plazola, R.A., R.M. Davis, and J.J. Marois. 2003. Effects of relative humidity and high temperature on spore germination and development of tomato powdery mildew (Leveillula taurica). Crop Prot. 22, 1157-1168. Doi: 10.1016/S0261-2194(03)00157-1 [ Links ]
Huber, L. and T.J. Gillespie. 1992. Modeling leaf wetness in relation to plant disease in epidemiology. Annu. Rev. Phytopathol. 30, 553-577. Doi: 10.1146/annurev.py.30.090192.003005 [ Links ]
Luo, W.H. and J. Goudriaan. 2000. Dew formation on rice under varying durations of nocturnal radiative loss. Agric. For. Meteorol. 104, 303-313. Doi: http://dx.doi.org/10.1016/S0168-1923(00)00168-4 [ Links ]
Magarey, R.D., J.M. Russo, and R.C. Seem. 2006. Simulation of surface wetness with a water budget and energy balance approach. Agric. For. Meteorol. 139, 373-381. Doi: 10.1016/j.agrformet.2006.08.016 [ Links ]
Mashonjowa, E., F. Ronsee, M. Mubvuma, J.R. Milford, and J.G. Pieters. 2013. Estimation of leaf wetness duration for greenhouse roses using a dynamic greenhouse climate model in Zimbabwe. Comput. Electron. Agric. 95, 70-81. Doi: 10.1016/j.compag.2013.04.007 [ Links ]
Mersha, Z., S. Zhang, and B. Hau. 2014. Seasonal dynamics of black leaf mould (Pseudocercospora fuligena) on greenhouse-grown fresh market tomatoes. J. Phytopathol. 162, 158-169. Doi: 10.1111/jph.12165 [ Links ]
Pedro Jr., M.J. 1981. Estimating dew duration. I. Utilizing micrometeorological data. Agric. Meteorol. 25, 283-296. Doi: 10.1016/0002-1571(81)90081-9 [ Links ]
Orlandini, S. and M. Rosa. 1997. A model for the simulation of grapevine downy mildew. Petria 7(Suppl. 1), 47-54. [ Links ]
Qian, T., J.A. Dieleman, A. Elings, A. de Gelder, O. Van Kooten, and L.F.M. Marcelis. 2012. Vertical temperature gradients in the semi-closed greenhouses: occurrence and effects. Acta Hort. 927, 59-66. [ Links ]
R Core Team. 2012. A language and environment for statistical computing. In: R Foundation for Statistical Computing, http://www.R-project.org/; consulted: November, 2015. [ Links ]
Rosa, M. and S. Orlandini. 1997. Structure and application of the PLASMO model for the control of grapevine downy mildew. Petria 7(Suppl. 1), 61-70. [ Links ]
Rosa, M., B. Gozzini, S. Orlandini, and L. Seghi. 1995. A computer program to improve the control of grapevine downy mildew. Comput. Electron. Agric. 12, 311-322. Doi: 10.1016/0168-1699(95)00007-Q [ Links ]
Sentelhas, P.C., T.J. Gillespie, J.C. Batzer, M.L. Gleason, J.E.B.M. Monteiro, J.R.M. Pezzopane, and M.J. Pedro Jr. 2005. Spatial variability of leaf wetness duration in different crop canopies. Int. J. Biometeorol. 49, 363-370. Doi: 10.1007/s00484-005-0259-1 [ Links ]
Sentelhas, P.C., T.J. Gillespie, M.L. Gleason, J.E.B.M. Monteiro, J.R.M. Pezzopane, and M.J. Pedro Jr. 2006. Evaluation of a Penman-Monteith approach to provide "reference" and crop canopy leaf wetness duration estimates. Agric. For. Meteorol. 141, 105-117. Doi: 10.1016/j.agrformet.2006.09.010 [ Links ]
Sentelhas, P.C., A. Dalla Marta, S. Orlandini, E.A. Santos, T.J. Gillespie, and M.L. Gleason. 2008. Suitability of relative humidity as an estimator of leaf wetness duration. Agric. For. Meteorol. 148, 392-400. Doi: 10.1016/j.agrformet.2007.09.011 [ Links ]
Tanner, C.B. and M. Fuchs. 1968. Evaporation from unsaturated surfaces: a generalized combination method. J. Geophys. Res. 73, 1299-1304. Doi: 10.1029/JB073i004p01299 [ Links ]
Wang, S., T. Boulard, and R. Haxaire. 2000. Measurement and analysis of air speed distribution in a naturally ventilated greenhouse. Acta Hort. 534, 277-283. [ Links ]
Wilcoxon, F. 1945. Individual comparisons by ranking methods. Biometrics Bull. 1, 80-83. Doi: 10.2307/3001968 [ Links ]
Wilks, D.S. 1995. Statistical methods in atmospheric Sciences. Academic Press, San Diego, CA. [ Links ]