I. INTRODUCTION
Limonite is an iron oxide hydroxide, whose general chemical formula is FeO(OH)-nH2O 1,2; it has variable quantities of goethite (FeOOH) and hematite (Fe2O3), and may be accompanied by certain accessory minerals that contain silicon, aluminum, phosphorus, and nickel, among other elements. Despite being a highly hydrated iron oxide, the limonite may be considered attractive to obtain metallic iron after the application of a heat treatment of calcination that modifies the structural arrangement of the chemically bonded water and the OH- groups, allowing its transformation into hematite above a critical temperature 2,3. The determination of this temperature, and the knowledge of the mineralogical changes undergone by the limonitic ore during a calcination process are of vital importance for the continuous improvement of the mineral beneficiation, and the metallurgical processes in both iron 3 and nickel industries 4.
Many iron-ore producing countries have been forced to assess the feasibility of using hydrated oxides and iron oxy hydroxides (goethite and limonite) as an alternative resource to obtain reduced iron. To be used economically, these minerals require a special reduction process due to their low iron content 5. Currently, many processes of mineral beneficiation have been devised for this type of ores. One of the current operational trends consists in increasing the temperature of the reduction zone, in order to attain a higher productivity, due to the thermodynamic improvement of the reaction conditions 6. For this reason, it has been suggested to evaluate the possibility of applying a heat treatment prior to the reduction stage, in order to reduce the moisture content of the ore, as well as its chemically bound water content 7; this procedure may also improve the reactivity characteristics of the ore, which can save processing time in the reduction stage. Another problem of limonitic ores is their strong tendency to present impurities; in fact, a typical case is the presence of phosphorous, which is a deleterious element, very difficult to control during the reduction and steelmaking stage. This feature strongly limits the use of phosphorous limonite ores to produce iron and steel. Nevertheless, these ores can be dephosphorized through a calcination process followed by leaching with acids or strong alkalis 3. In this case, it is required to know how the solid is affected by the calcination process, since its final structure will modify its specific surface area, which in turn, will sign the effective leaching of phosphorus, increasing the efficiency of the hydrometallurgical beneficiation process 3. In summary, the advantages of applying a calcination treatment as a preliminary stage of the reduction process are based on improving the efficiency of the reduction process itself, and the possibility of reducing or eliminating the presence of undesirable elements in the reduction reactors. Additionally, the amount of ore entering the reactors during the production process would be reduced, facilitating, in such a way, the handling and transportation of materials within the facility.
Currently, the Venezuelan iron ore industry faces a critical situation, in which the premium quality minerals are running out, resulting in an enrichment of the exploitation mining fronts with low-grade limonitic fractions that also have higher phosphorus content. This situation is common in other parts of the world, such as Australia 7, and implies major challenges for the industry, in terms of mineral handling and further processing. For this reason, it is necessary to conduct an exploratory research to understand the behavior of this type of limonitic ores during calcination. Based on the afore-mentioned situation, this study was carried out with the aim of evaluating the effect of a heat treatment of calcination at different temperatures on the crystal structure of two limonitic iron ores from southern Venezuela. To reach this objective, we applied several analytical techniques, including thermogravimetry (TG), X-ray diffraction (XRD), and Fourier transform infrared spectroscopy (FTIR).
II. EXPERIMENTAL METHODOLOGY
A. Minerals tested
Two limonitic iron ores (locally known as "limonitic crusts") were obtained from "Los Barrancos" and "Cerro Bolívar" iron ore deposits, both located in the Bolivar State, in southern Venezuela. Before all the tests were made, the ores were crushed to a particle size smaller than 90 urn using an agate mortar.
B. Experimental work
1) Thermogravimetric analysis (TGA): For the analysis, a CAHN/VENTRON thermobalance (precision 10 mg) was used. A 100 ± 10 mg sample of iron ore was placed in a 9-mm diameter platinum basket that was connected to the thermobalance through a quartz thread. The set was placed inside a GORDON® cylindrical convection oven, preheated to the required temperature. Voltage was measured and recorded every 1/15 seconds until it reached a constant value. With the aim of knowing the mass loss behavior of the mineral as a function of the temperature, this test was repeated at different temperatures from room temperature to 950 °C, using a heating rate of 10 °C/ min.
2) X-Ray diffraction analysis (XRD): The X-Ray diffraction analysis was carried out on original condition samples (no heat treatment), and on treated samples in a muffle furnace during one hour at the following temperatures (°C): 250, 350 and 950. The diffractograms were obtained with a diffractometer Philips XPERT Pro, with a Co Ka radiation (wave length λ=1.78897 Â), in a range between 5°<20<9O°. A voltage of 40 kV, a current of 20 mA, and a scanning rate of 0.02 20/s were used. The different phases present in the sample were identified through a detailed comparison with the goethite and hematite files, contained in the database of the PDF-ICDD (Powder Diffraction File - International Centre for Diffraction Data).
3) Fourier-Transform Infrared Spectroscopy Analysis (FTIR): This analysis was carried out on both iron ores with and without heat treatment for one hour, in a temperature range between 250 °C and 950 °C (at 100 °C intervals). The FTIR spectra were obtained with a BRUKER TENSOR 7 spectrometer, and were recorded and standardized through baseline corrections and atmospheric compensation. The equipment was calibrated using a potassium bromide (KBr) pellet. Subsequently, sample pellets diluted in KBr were made using a small portion of sample (mass ratio 99:1).
III. RESULTS AND DISCUSSION
A. TGA Analysis
We determined the mass loss during the calcination process of the studied minerals by means of a TGA analysis. The diagrams in figures 1 and 2 show that as the temperature increases, the percentage of mass loss progressively increases, with a greater mass loss observed at applying the calcination heat treatment at 950 °C. The dehydration of limonite involves the loss of free water, chemically bound water, and hydroxyl groups that form part of the goethite structure [8, 9], to finally obtain the hematite structure. This process is described by means of the global reaction shown in equation (1).
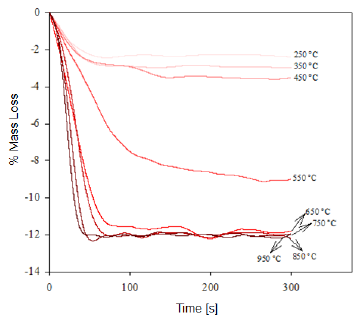
Fig. 1 Thermogravimetry of the "Los Barrancos" iron ore deposit, representing the mass loss of the samples in function of the time for the specified calcination temperature.
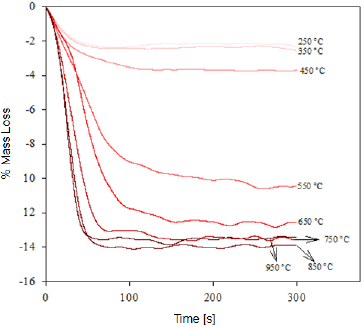
Fig. 2 Thermogravimetry of the "Cerro Bolívar" iron ore deposit, representing the mass loss of the samples in function of the time for the specified calcination temperature.
This dehydration process comprises three stages (Fig. 1 and 2): The first stage occurs at temperatures between 250 °C and 350 °C, and it is basically related to the moisture loss in the mineral; the second stage, in the temperature range of 450-550 °C, is related to the loss of chemically bound water; and the last stage, at 550 °C, is related to the loss of OH- groups of the goethite structure, forming hematite (Fe2O3), as shown in equation (2) 8,9.
It is estimated that the loss of free water occurring during the first dehydration stage, due to the heat treatment at low temperatures, can be explained by the fact that this water is either absorbed on the surface or occluded into the microscopic pores of the iron ore microstructure [8, 9]. In addition, during the last stage of calcination, large amounts of OH- groups are emitted out as water vapor from the remnant goethite or from the initially formed hematite, the latter still containing hydroxyl groups in its structure 8-11. Such functional groups would be combined as a result of the atomic movement induced by the high treatment temperatures. The slope changes observed in figure 3 are due to the different ways in which the water may be present in the samples.
At the beginning of the dehydration process, i.e. at temperatures below 250 °C, the water absorbed by the mineral is rapidly removed [10]. Once this temperature is exceeded, goethite must have completed its phase transformation process, becoming hematite, which was proven through the XRD (Fig. 4 and 5) and FTIR (Fig. 6 and 7) analyses. However, the structure of the newly-formed hematite still contains an excess of hydroxyl units [10]. For this reason, we did not observed a major change in the percentage of mass loss in any of the iron ores (Fig. 1 and 2), where the heat treatment was applied to 250 °C.
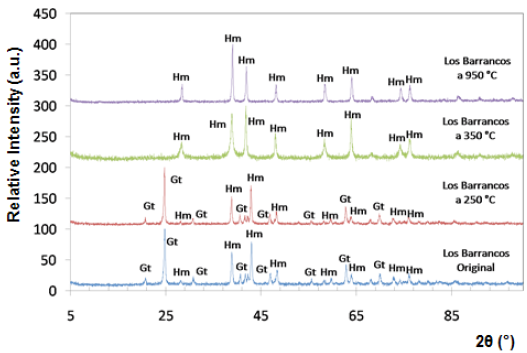
Fig. 4 Diffractograms of limonitic iron ore from "Los Barrancos" deposit, before heat treatment. Radiation Ka-Co (range 5°-90°).
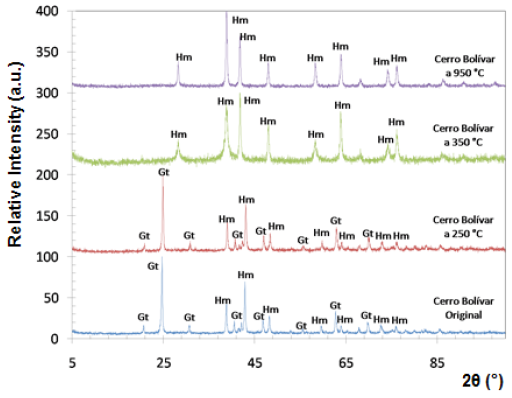
Fig. 5 Diffractograms of limonitic iron ore from "Cerro Bolivar" deposit, before heat treatment. Radiation Ka-Co (range 5°- 90°).
As the temperature rises, the reaction preferably starts in the places where the surface per unit volume is higher. Because the hematite has begun to emerge on the goethite surface, the process does not continue progressively, since this last phase is occluded by the newly-formed hematite, and therefore, it will be necessary to increase the temperature so that dehydration of the mineral continues 9. Furthermore, as temperature increases, so does the pressure of the water contained inside the mineral, resulting in the formation of internal microporosities 10. On that basis, since the diffusivity of Fe3+ and O2- is rather slow 9, it can be assumed that this is the reason why we did not observed a major change in mass loss % for both minerals, in the range between 250 °C and 350 °C.
As shown in Figure 3, a significant increase in the slope occurred at temperatures between 300 °C and 450 °C; this increase corresponds to the sustained increase in pressure of the water contained into the mineral micropores, which makes them keep growing and subsequently join together 10. This behavior continues until around 650 °C, temperature at which the coalescence of micropores generates macroporosities, which will facilitate the complete removal of the remaining water within the mineral particle 10,12. With the generation of this new area at high temperature, the diffusion effects begin to take preponderance and Fe atoms can migrate, starting to adopt the shared octahedral arrangement that leads to the more condensed crystallographic phase of the hematite 12.
When the heat treatment is carried out at about 700 °C, there is an even greater mobility of the atoms in the crystal lattice, which leads to a further increase in the diffusional phenomena, whose outcome is a higher mass decrease in both samples, due to atomic rearrangement 10. At 950 °C, the atomic movement within the crystal structure finishes, and the formation of the hematite is completed, accompanied by a decrease in anisotropy and the generation of an ordered structure 9.
B. X-Ray Diffraction Analysis
Both iron-ore samples were characterized by X-ray diffraction, in their original condition and after being treated at different temperatures. The two main crystalline phases revealed in the original state of the material corresponded to goethite (Gt) and hematite (Hm), as shown at the bottom of figures 4 and 5. Goethite has an orthorhombic structure (lattice parameters: a = 9.95 Â, b = 3.01 Â, c = 4.62 Â), while the hematite has a rhombohedral hexagonal structure, similar to corundum (lattice parameters: a = 5.035 Â, c = 13.752 Â) 13.
Within the analyzed diffraction range, eight peaks associated with hematite, and eight with goethite were detected. It is presumed that goethite is present in higher proportion than hematite, because the peaks associated with goethite show a higher relative intensity.
The X-ray diffraction technique allowed to follow the changes that took place over the crystalline structure of limonitic minerals due to the increase in time and temperature of the heat treatment. Figures 4 and 5 show the diffractograms obtained for all temperatures during one hour of treatment. Between 250 °C and 350 °C, we observed a change in the diffraction peaks of the studied minerals, characterized by the disappearance of some of the peaks associated with goethite, which were present before the heat treatment and in the sample treated at 250 °C. New peaks associated with hematite also appeared at temperatures above 350 °C.
At room temperature and at 250 °C, the peaks of goethite prevailed in the structure, whereas from 350 °C, all peaks associated with goethite disappeared, and the hematite peaks were revealed, demonstrating that a re-arrangement occurred in the atomic positions of the goethite crystal lattice, thus being transformed into hematite. For both studied minerals, the transformation range was between 250 °C and 350 °C. This transformation is favored by the structural links existing between certain planes of the hematite and the goethite structures 14.
Several theories have been proposed to study the transformation of goethite to hematite at high temperatures. The heating causes dehydration of the goethite, resulting in slight modifications of its crystal structure. Transformation begins on the surface of the goethite, and then advances into the mineral, forming multiple microporosities, as it was deduced from the thermogravimetric analysis. Transformation may involve one or two intermediate stages of hematite 15. The first stage (protohematite) is formed as a consequence of heating between 250 °C and 400 °C, while the second stage (hydrohematite) appears at heating temperatures above 430 °C. Above 800 °C, the structure of the relatively ordered hematite is obtained 16. Some authors revealed that one of the main characteristics of the intermediate phases is the great similarity of their maximum diffraction peaks with those of the hematite 11,15. In this sense, Schwertmann 8 found that the different forms of hematite formed during the dehydration of goethite have a defective structure, which is characterized by the widening of the diffraction peaks. However, although the existence of such intermediate stages is not part of the scope of this paper, it is evident that many investigations support that a transformation of goethite to hematite occurs during heating at elevated temperatures.
C. Fourier-Transform Infrared Spectroscopy Analysis (FTIR)
Analysis by Fourier-Transform infrared spectroscopy allowed studying the behavior of the functional groups present in the mineral. Figure 6 shows the infrared spectrum of limonitic iron ore, from the "Los Barrancos" deposit, with no heat treatment. In the spectrum, seven predominant bands can be noted: Four of them associated with stretching and strain vibrations of hydroxyl groups (A, D, E), two of them associated with water bending vibrations (B, C), and the last two bands related to the vibration of the Fe-O bond in the crystal arrangement of goethite and hematite (F, G) 16. Figure 7 shows the bands for the limonitic iron ore from the "Cerro Bolívar" deposit, which were identified by the same letters, but with an apostrophe symbol (').
As mentioned above, it is very common for goethite to exhibit an excess of hydroxyl groups within its crystalline structure. Therefore, the structure of this mineral favors the presence of the OH vibrations in the FTIR spectrum. Its structure is composed of a compact hexagonal arrangement of oxygen atoms, like most of the iron oxides and oxyhydroxides 8,14. The location of each atom within the mineral crystal lattice favors the formation of hydrogen bonds in two different directions, facilitating the presence of some hydroxyl groups in the structure 14. For this reason, the infrared spectra of limonite show the stretching and strain vibrations of hydroxyl groups, which basically depend on the direction and strength of the atomic bond that characterizes these groups.
By way of comparison, it is observed that the bands associated with goethite, those related to water and OH groups vibrations, have a higher intensity in the case of the "Cerro Bolívar" ore (Fig. 7). On that basis, the assumption made earlier about the presence of more hydroxyl groups in this mineral can be verified, since it is expected that the transmittance intensity in that region of the spectrum is proportional to the concentration of functional groups within the structure [12]. It should also be noted that the spectra shown in figures 6 and 7 exhibit subtle spectral perturbations in the region between 1080 cm-1 and 1125 cm-1, forming shoulders (Fig. 6) or small peaks (Fig. 7), which correspond to vibrations of Si-O bonds, indicating the minority presence of quartz or silicates within the natural goethite 18.
On a preliminary basis, both iron ores present a similar dehydration process (Fig. 8 and 9), going through the same structural changes as the calcination temperature increases. The hematite formed from the goethite can inherit both the excess hydroxyl units and the structural characteristics of the initial goethite, as a consequence of heat treatment at low temperatures (around 300 °C), which help to distinguish the dehydroxylation process that is carried out during the thermal transformation 14. In the following sections, we analyze in detail the behavior of the hydroxyl units in relation to the changes on the position and intensity of the FTIR spectral bands, during the thermal transformation of goethite to hematite observed in both limonite crusts obtained from the Venezuelan "Los Barrancos" and "Cerro Bolívar" iron-ore deposits.
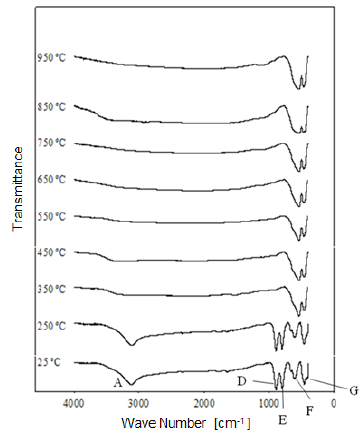
Fig. 8 Modification of the infrared spectrum of iron ore from "Los Barrancos" deposit, after being heat treated at different temperatures for one hour. 25 °C refers to the sample without heat treatment.
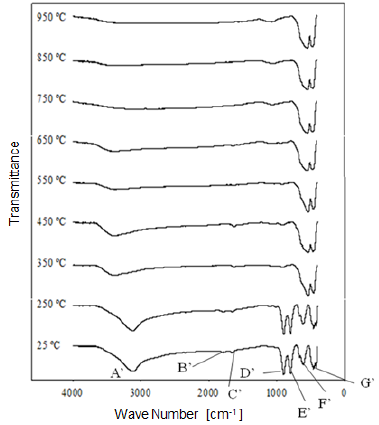
Fig. 9 Modification of the infrared spectrum of iron ore from "Cerro Bolívar" deposit, after being heat treated at different temperatures for one hour. 25 °C refers to the sample without heat treatment.
1) Stretching vibration of O-H groups: The first spectral band detected in the ore from "Los Barrancos" deposit is located in a frequency range of 3588 cm-1 to 2861 cm-1, while for the ore from "Cerro Bolívar" deposit, is between 3603 cm-1 and 2765 cm-1. Both values are associated with stretching vibrations of the O-H bonds 17. This band is very sensitive to the dehydroxylation temperature, and is one of the most important bands to be considered for the characterization of the thermal transformation process of goethite 14.
The position of this center band moves toward higher wave numbers as the calcination temperature increases until reaching 350 °C, since at temperatures above this value, the band stops showing peak shape to become a small shoulder that disappears above 450 °C, in the case of the "Los Barrancos" ore (Fig. 8), and above 650 °C, in the case of the "Cerro Bolívar" ore (Fig. 9). The first displacement of this band occurs at 250 °C, suggesting the beginning of the structure alteration (from goethite to hematite), and as the band moves further, the energy of the hydrogen bonds decreases 16, due to the increase in the angle formed by the atoms in the O-H...O bond inside of the atomic arrangement of the mineral 13. This occurs because some of the atoms of the crystal lattice are attracted to neighboring OH ions, which results in a weakening of the hydrogen bond that connects the OH ions to the crystal lattice 16.
Between room temperature and 350 °C, in addition to the band displacement, there is a decrease in its intensity and bandwidth, which is a characteristic change that occurs when the hematite structure has been completely formed 14. However, this structure will retain an excess of OH groups, which generates a shoulder-shaped signal in this area of the spectrum that will not disappear until higher calcination temperatures are reached 14.
2) Bending vibrations of the O-H groups: The region between 1840 cm-1 and 1615 cm-1 (Fig. 8 and 9) shows two small bending bands associated with water, which are designated as S'(OH) and g'(OH). The lowest intensity observed in the bending bands is due to the fact that they have low sensitivity when are irradiated using infrared light 17. On the other hand, the behavior of these bands during the dehydration process is equivalent to that shown by stretching and strain vibrations of the hydroxyl groups 14,17. Similarly, such bending bands are no longer present in the infrared spectra after calcination at 350 °C (Fig. 8 and 9).
3. Deformation vibrations of the O-H groups: The spectrum range between 978 cm-1 and 852 cm-1 shows two very marked bands that correspond to the deformation of the hydroxyl groups, and are called S(OH) and g(OH). The division of these bands is the result of the two different ways in which hydroxyl groups that are not part of the mineral stoichiometry may be deformed 17. These bands, called D, E and D', E' respectively, have a shape similar to a "W" (Fig. 6 and 7), which is characteristic of goethite 12,18. The first one, the d(OH) band, is attributed to the atomic vibration in the mirror plane of the goethite (plane a-b), while the second one, the g(OH) band, is essentially related to the vibrations occurring outside of this plane 17. The axes corresponding to the goethite structure are presented in figures 10, 11, and 12.
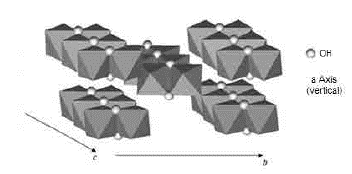
Fig. 10 Axes a, b, and c of the goethite 8. Double octahedron chains are indicated. The unmarked vertices of the octahedron correspond to oxygen
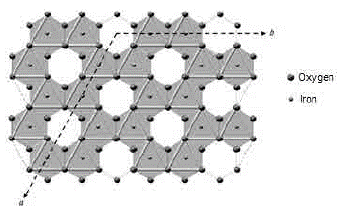
FIG. 11 Axes a and b of the hematite 8. An octahedral vacancy is indicated for every two octahedrons of Fe3+ in the center
The resulted spectra show that these bands exist in the original state of the ore, and at the dehydroxylation temperature of 250 °C (Fig. 8 and 9). In addition, there is no significant change in the displacement of the band centers, which is related to the fact that there is no change in the distance or bonding energy of the functional groups, compared to the bands associated with stretching vibrations 17. On the other hand, the early disappearance of these bands with respect to the others is due to the fact that the hydroxyl groups can be released easily from the goethite planes, since its structure is usually more defective; these structural defects contain more OH groups, which favors the release of hydroxyls during calcination 17.
4) Own vibrations of hematite: The characteristic bands of hematite, between 650 cm-1 and 406 cm-1, are related to the Fe-O vibration within the crystal structure of iron oxides and oxyhydroxides. In the studied ores, these bands were observed at lower frequencies. The first ones that appeared in the spectra are attributed to the vibration of the Fe-O bond in the a-plane of the hematite, while the others are associated with the vibrations of such bond in the plane c of the hematite (Fig. 11 and 12).
In general, the bands associated with plane a are not very defined in the goethite infrared spectrum 12. However, their appearance in the spectrum from the untreated samples is attributed to the fact that the studied iron ores are composed of a mixture of goethite and hematite (Fig. 4 and 5). The largest change observed in the spectra of figures 8 and 9 occurred between 250 °C and 350 °C, which corroborates the explanation that the above range corresponds to the structural transition temperature 12,14, during which goethite is converted into hematite. Additionally, in this area of the spectrum, a displacement to lower frequencies of the band related to the hematite plane c was observed; such a displacement occurred due to the appearance of a new hematite phase after calcination 14. For both limonitic iron ores, above 350 °C, the band center associated with the planes a and c of the hematite remained in the same position as the treatment temperature increased, which indicates that there is no change in the Fe-O bond distance in the newly formed hematite structure 17.
IV. CONCLUSIONS
Limonitic iron ores of the "Los Barrancos" and "Cerro Bolívar" deposits exhibited a decrease in mass due to the loss of both free and structural water (chemically bound or in the form of OH groups), after being submitted to a calcination heat treatment at different temperatures between 250 °C and 950 °C.
After characterizing samples using X-Ray diffraction, it was found that a modification of the mineral structure occurs between 250 °C and 350 °C. The structure, which was constituted of two mineralogical phases (goethite and hematite), was completely transformed into hematite as a result of the calcination process at temperatures above 350 °C.
The results obtained using FTIR spectroscopy showed a similar range of transformation to that obtained by X-Ray diffraction. At temperatures above 350°C, a modification occurred in the infrared spectra, exhibiting the disappearance of the stretching and deformation bands of the OH groups, the disappearance of the water bending bands, and the displacement of Fe-O vibrations (of the hematite axis c) toward lower frequencies. However, despite the transformation of phases to hematite, the transformed ore still maintained a certain amount of OH groups, even at higher calcination temperatures.
Obviously, such structural modifications facilitate the mass loss manifested during the calcination process. In this respect, one advantage is that the mass loss occurred during calcination of iron ores prior to the reduction process could reduce the amount of charge introduced into reactors during the production process, and could facilitate the production of a more reactive ore during the reduction process.