I. Introduction
The current technological revolution and the appearance of low-cost and high-performance electronic components have allowed the design and manufacture of embedded devices for the fulfillment of specific tasks. In the health sciences case, there is an increasing number of technological platforms that enable the clinically objective diagnosis of patients [1, 2] and host another kind of applications [3,4,5]. When the medical approach plays a prominent role in the measuring of the human body parameters, cutting-edge technology such as wearable or embedded devices are essential to aid diagnostic and rehabilitation processes [6,7,8], although in some cases they have affordability and/or accessibility limitations. In general, systems that capture the mechanical movement of the human body are used in different diagnostic processes [9, 10] and are based on various technologies [11,12,13]. Within this group, those systems built with magnetic and inertial sensors stand out due to their low-cost and high-performance technical features. However, in many cases, it is not possible to send the collected data in real-time, which is a limitation regarding the user-friendliness of the devices for complex capture environments, as regards the data acquisition and centralization.
This paper presents a chronological literature review to identify and recognize the different technologies that make up the wireless communication used for biomechanical motion characterization. At the same time, the architecture of the Imocap signal acquisition platform, developed by the GIS Software Research Group of the Pedagogical and Technological University of Colombia, is described. This platform integrates the most robust characteristics of an inertial-magnetic system and the data transmission in real-time via Wi-Fi.
To contextualize the main elements addressed in this research, the information below exposes aspects such as the method used to review the literature, relevant works on the use of wireless networks in the medical field and general information on wireless motion capture systems based on inertial and magnetic technology.
Among the most representative developments, there are several works focused on the monitoring equipment at hospitals: In the review by Malasinghe et al. [14] an overview of the monitoring systems connected to patients in hospitals is presented, where the vast majority of the prototype or architectures presented use wireless communications. Abreu [15] focused on the service quality of wireless sensor networks in intensive care units. Bocicor [16] proposed a prevention system for hospital-acquired diseases through wireless sensor monitoring, and finally, Iancu [17] presented a method to linking medical devices to a wireless network, even when they have not been designed for this.
Other developments focus on the efficient wireless transmission data or its associated power consumption [18,19,20], since most medical applications require energy-efficient portable systems. From the information quality standpoint, the Sharma [21] and Casilari-Pérez [22] works stands out in the medical field, as they detected anomalies in signal transmissions in medical applications. Inherent in the efficiency of wireless data transmission, other works have evaluated and identified a better way to access data in medical applications [23, 24].
To conclude this section, it is relevant to mention the technology used in rehabilitation and diagnosis [25,26,27,28], in which the active monitoring of patients in different conditions and the wireless data transmission with different types of technology predominate.
For the biomechanical motion capture, wireless networks can be used as a detection method or as a means of data transmission [29,30,31,32,33]. Likewise, other developments like [34] show the measurement of different biomechanical parameters using a MEMS-based accelerometer and wireless data communication based on Bluetooth, or the acquisition of biomechanical signals and their processing in real-time [35].
Several applications use Zigbee and/or Bluetooth wireless transmission technology, as is the case of a health monitoring system for remotely located patients [36], a motion measurement system to help patients with motor problems [37], the monitoring of different diseases that may affect limb motion [38], among other works [39,40,41].
Finally, some papers document biomechanical motion capture systems based on inertial-magnetic sensors, including a Wi-Fi and Zigbee wireless communication system. In [42], a capillary oxygen saturation and motion monitoring device is presented, using a system for capturing and transmitting data through Zigbee. Supplementary development is presented in [43], in which a robot is controlled with biomechanical gesture recognition, capturing the data with MEMS devices. In the same way, there are other developments [44,45,46,47] that address this issue and use Wi-Fi technology.
This literature review highlights the electronic devices development focused on medicine or rehabilitation that use wireless communication, mainly applying radiofrequency-based technologies (RF), and more often Zigbee, where not all of them use Wi-Fi despite the advantages that this technology offers. Additionally, the developments in motion capture systems based on inertial-magnetic technology are scarce considering the integration of real-time data transmission through Wi-Fi wireless communication. Due to this situation, conducting this research is useful since it integrates three main elements: 1) a technology that has not been widely explored in the biomedical area, as is the use of Wi-Fi; 2) the use of inertial-magnetic sensors that allow the capture of biomechanical data; and 3) real-time data transmission which is processed and collected by the system.
II. Materials and Methods
This section describes the technical and operational characteristics of the Imocap motion capture system, including the connection to the peripheral devices.
A. Platform Architecture
The Imocap motion capture platform consists of several components. The most important ones are described below.
Central Processing Unit (CPU). Provides the fundamental logical support for biomechanical data capturing, processing and communication.
Wi-Fi ESP8266 Wireless Communication System. A wireless communication central module that allows for the transmission and data reception. It is a core element for the development of this research.
Peripheral ports. Allows the addition of MEMS-based motion capture sensors.
Memory card. Stores the biomechanical data in removable media when the wireless communication module is not in use.
Battery. Provides electrical power to the system, which offers 67 minutes of autonomy at maximum wireless output power and 100% CPU usage.
B. Connection of Devices Through Imocap
Through the peripheral ports, Imocap can communicate with different devices such as sensors and actuators, both analog and digital. This ability to interact with other devices makes Imocap a robust process unit that allows the generation, analysis and transmission of biomechanical signals. To carry out this research, a motion processing unit (MPU) was wired to one of the Imocap's peripheral ports. The MPU is a combination of sensors manufactured with MEMS technology designed for motion acquisition, providing real-time information and data at a maximum rate of 1000 samples per second (samples/s). For this study, the MPU sent motion data including also Euler angles that provide consolidated information at 30 samples/s rate.
C. Biomechanical Data Collection Technique
The data provided by the MPU through Imocap allow the reconstruction of the biomechanical movement when the system is placed in the human body. Bearing in mind that the approach of this research is to demonstrate that the use of a Wi-Fi-based communication is efficient for the transmission and collection of biomechanical signal data, there is no specific protocol for the collection of such motion information. Since it is necessary to determine whether the wireless data transmission is interrupted or damaged, the Imocap system will also record the biomechanical signals on the integrated memory card.
D. Test Protocols
For wireless data transmission, an ESP-12F card based on ESP8266 is used, which includes a 32-bit microcontroller with a 160 MHz clock and an integrated antenna [48]. Concerning the operating modes of this communication system, Soft Access Point (AP) and Station (STA) were used.
1) Spectrum Analysis for Wi-Fi Communication at 2.4GHz. Since wireless data communication is done through Wi-Fi, the indoor, as well as an outdoor test environment is contaminated by other Wi-Fi networks. Therefore, there is a preliminary inspection of the communication spectrum in the 2.4 GHz frequency band in both AP and STA modes. The spectrum analysis helps in the preliminary identification of whether the signal saturation in the test environment is high, which is a direct cause of possible difficulties for the transmission of biomechanical data.
2) Indoor Data Transmission Test. The Imocap device was initially tested indoors, since most biomechanical data collection applications are carried out in laboratories, medical centers, testing rooms or homes. Thus, for indoor tests, where protocol 1 uses the AP mode and protocol 2 uses the STA mode, a biomechanical motion collection of a human back was made while a person walked through a closed space, walking naturally through the different areas. The parameters to be evaluated in the development of the indoor test protocols are: transmission signal strength towards the receiving device in decibel-milliwatts (dBm), communication speed in megabytes per second (MB/s), latency in milliseconds (ms) and the data quality percentage (%), comparing the information received via Wi-Fi versus that stored locally in the memory card within the system.
3) Outdoor Data Transmission Test. The Imocap device has also been tested outside the laboratory, as some of the applications for biomechanical evaluation are performed in open spaces. A clear example of this situation is the biomechanical analysis of an athlete while performing physical activity.
In the outdoor tests, protocol 3 used the AP mode and protocol 4 used the STA mode, and a biomechanical collection of the back movement was carried out while the person walked in a straight line along a residential access road, with a line-of-sight to the measurement point.
The parameters to be evaluated in the development of the outdoor test protocols are: transmission signal strength towards the receiving device in decibel-milliwatts (dBm), communication speed in megabytes per second (MB/s), latency in milliseconds (ms), noise ratio (%) and data quality (%), comparing the data received through Wi-Fi to the one stored locally in the memory card within the system.
III. Results
Below are the results obtained after the application of the test protocols explained in the previous section.
A. Results of the Spectrum Analysis for Wi-Fi Communication at 2.4GHz
The results of the frequency spectral analysis are presented below.
In Figure 1, it can be seen that there are 15 wireless networks near the Imocap system, which can cause radio frequency (RF) interference when this device is used in AP mode. Specifically, for channel 6, four wireless networks coexist and congest the same frequency: 2437 MHz. Even knowing that problems can occur in the effective data transmission, the protocol tests were carried out in that environment, demonstrating the “worst-case scenario”.
The transmission strength of the Imocap device is -43.5 dBm, measured by a receiver located at 10cm from the origin. In Figure 2, the frequency spectrum analysis is repeated, but using a ZTE ZXV10-W300 router as an Access Point, which will emit the Wi-Fi signal with “GIS_SoftwareResearchGroup” SSID. The Imocap device is linked to this signal in STA mode. The results shown are analyzed from the Imocap system.
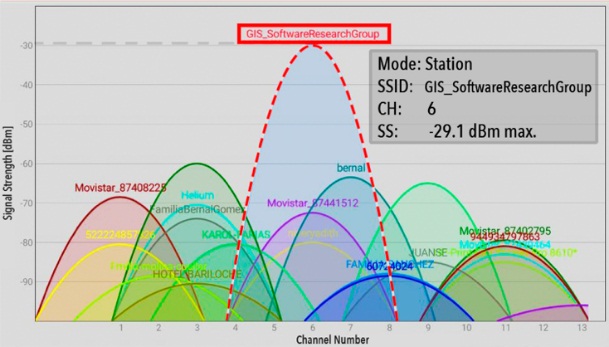
Fig. 2. Wi-Fi Spectrum Analysis for the test environment, using router as Access Point and Imocap as Station.
When Imocap is used in STA mode, the reception strength is -29.1 dBm at 10cm from the router. This indicates that there is a stronger signal strength when the router is used to support the network. About the saturation of the RF spectrum, the same case described in Figure 1 occurs.
B. Protocol 1: Indoor Test Using Imocap in AP Mode
For the indoor system assessment, a space with multiple solid divisions was chosen, similar to a laboratory in a specialized medical center. In the first trajectory, the Imocap system (only one) is functioning in AP mode, emitting the Wi-Fi signal and moving through the evaluation area. This movement in the indoor space was realized slowly and maintaining a constant rhythm. To have a better understanding of the Wi-Fi signal dissipation produced by the Imocap device, a heat map is presented in Figure 3.
For this indoor test, a total of 81.25 m2 were covered, distributed as a rectangle in space. It is important to note that the measurement point is an Apple MacBook Pro laptop, which remained static throughout the test. In Figure 3, is noted there is a strong signal in the main work area (adequate level) descending to weak level in some cases, depending on the Imocap localization. When the device moved away from the measurement point, the signal lost intensity, which affected the data transmission performance. The remaining evaluation parameters are recorded in Table 1.
Table 1 Using Imocap as AP Protocol 1 Indoor Test
Strong signal (between 0 and -50 dBm) | |
Max. Signal Strength | -43.5 dBm |
Avg. Signal Strength | -48.6 dBm |
Max. Transmission Speed | 2.73 MB/s |
Avg. Transmission Speed | 2.30 MB/s |
Avg. Latency | 6 ms |
Data Quality (Effective data reception) | 100% |
Adequate signal (between -50 and -70 dBm) | |
Avg. Signal Strength | -67.9 dBm |
Max. Transmission Speed | 2.68 MB/s |
Avg. Transmission Speed | 2.17 MB/s |
Avg. Latency | 8 ms |
Data Quality (Effective data reception) | 98% |
Weak signal (between -70 and -90 dBm) | |
Avg. Signal Strength | -78.3 dBm |
Max. Transmission Speed | 1.98 MB/s |
Avg. Transmission Speed | 1.73 MB/s |
Avg. Latency | 13 ms |
Data Quality (Effective data reception) | 87% |
From Table 1, it can be concluded that the Imocap system has enough coverage for small spaces in the presence of obstacles, such as concrete walls and RF interferences from nearby wireless networks. It is considered that the wireless transmission is acceptable for biomechanical signals, even when strength levels under -70 dBm are reached, where the information reception percentage is around 87%. Latency, in any case, does not represent an obstacle as its values remain low. It is shown that it is possible to use the Imocap system in AP mode, given that even with high interference and obstacles in the test room, it offers optimal performance, with a strong and adequate signal.
C. Protocol 2: Indoor Test Using Imocap in STA Mode
The procedure carried out in protocol 1 is repeated, but using Imocap in STA mode instead, walking along the test area and sending the data to the main router. To show the Wi-Fi signal dissipation received by the Imocap system, a heat map is presented in Figure 4.
For this indoor test, a total of 81.25 m2 was covered. It is important to clarify that the measurement point used was the Imocap system itself, as it received the signal from a ZTE ZXV10-W300 router AP. The data was transmitted from the router to an Apple MacBook Pro Laptop through the network. According to Figure 4, the result obtained regarding the Wi-Fi network dissipation is similar to that found in figure 3, with the difference that the emitted signal strength is higher and more uniform due to the router range.
Table 2. Using Imocap as STA. Protocol 2: Indoor Test.
Strong signal (between 0 and -50 dBm) | |
Max. Signal Strength | -25.7 dBm |
Avg. Signal Strength | -42.8 dBm |
Max. Transmission Speed | 2.81 MB/s |
Avg. Transmission Speed | 2.32 MB/s |
Avg. Latency | 8 ms |
Data Quality (Effective data reception) | 100% |
Adequate signal (between -50 and -70 dBm) | |
Avg. Signal Strength | -53.7 dBm |
Max. Transmission Speed | 2.78 MB/s |
Avg. Transmission Speed | 2.28 MB/s |
Avg. Latency | 15 ms |
Data Quality (Effective data reception) | 99% |
Weak signal (between -70 and -90 dBm) | |
Avg. Signal Strength | -70.4 dBm |
Max. Transmission Speed | 2.16 MB/s |
Avg. Transmission Speed | 1.93 MB/s |
Avg. Latency | 27 ms |
Data Quality (Effective data reception) | 82% |
From Table 2, it is concluded that the router provides the Imocap system with a good wireless range, resulting in a higher overall signal strength versus Imocap in AP mode. The data transmission through the router to the final device causes the latency to increase considerably, but at the same time there is a benefit: to have a more stable communication, with an effective data reception of 82% in the worst-case scenario. It is concluded that the transmission test of biomechanical data indoors is effective, since the data reception performs correctly in either of the two modes, under non-ideal transmission conditions. Table 3 shows a general summary of the tests carried out in protocols 1 and 2.
Table 3. Indoor Test Summary.
Mode / Parameter | Imocap in AP mode | Imocap in STA mode |
Max. Signal Strength | -43.5 dBm | -25.7 dBm |
Avg. Signal Strength | -64.9 dBm | -55.6 dBm |
Max. Transmission Speed | 2.73 MB/s | 2.81 MB/s |
Avg. Transmission Speed | 2.07 MB/s | 2.17 MB/s |
Avg. Latency | 9 ms | 17 ms |
Avg. Data Quality | 95% | 97% |
Effective Area | 81.25 m2 | 81.25 m2 |
D. Protocol 3: Outdoor Test Using Imocap in AP Mode
For the system evaluation in an outdoor environment, a residential access road was used as a case study. The first outdoor path was performed using the Imocap system as AP, moving through the selected area and sending the data to the receiver. To show the Wi-Fi signal dissipation emitted by the Imocap system, a heat map was developed and is presented in Figure 5.
In this case, the measuring point used was an Apple MacBook Pro laptop, which remained static during the test. Besides, by having a straight-line path with line-of-sight, a signal strength ratio was established for each meter covered. In Figure 6, it is possible to uncover the relation between the distance and transmission strength, while in Table 4 there is a results summary of this protocol.
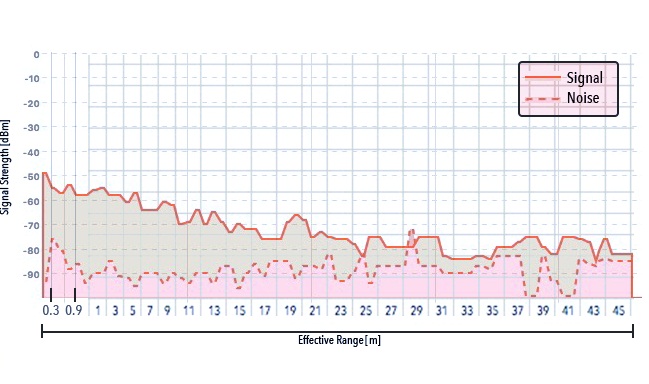
Fig. 6. Outdoor wireless signal characterization: signal strength and noise versus distance, using Imocap as Soft Access Point.
Table 4. Summary of the Protocol 3: using Imocap as Access Point, outdoor test.
Distance [m] | Avg. Signal Strength [dBm] | Noise [%] | Avg. Trans. Speed [MB/s] | Avg. Latency [ms] | Data Quality [%] |
0.3 | -55.0 | 11 | 2.16 | 6.34 | 100 |
0.6 | -56.5 | 5 | 2.02 | 6.26 | 100 |
0.9 | -57.3 | 2 | 2.04 | 6.22 | 98 |
1 | -58.6 | 5 | 1.96 | 6.48 | 98 |
5 | -62.4 | 3 | 1.83 | 6.81 | 95 |
11 | -64.7 | 9 | 1.87 | 7.29 | 92 |
21 | -70.1 | 11 | 1.63 | 8.24 | 77 |
43 | -84.1 | 13 | 1.39 | 9.53 | 70 |
45 | -83.0 | 18 | 1.46 | 9.62 | 70 |
The weather conditions were favorable for the experiment: average temperature 16ºC, no rain, relative humidity 72% and winds of 4 km/h. It can be concluded that the effective range of the biomechanical data capture system Imocap in AP mode is 45.6 m. Considering the total length of the access road, as well as the presence of interferences caused by the electromagnetic noise of the transmission lines and the presence of other wireless networks in the area, the maximum effective range is outstanding. In the worst-case scenario and at maximum distance, 70% of the information was received effectively, with an 18% noise and interference factor, the maximum transmission speed of 1.46 MB/s as a minimum and 9.62 ms latency.
E. Protocol 4: Outdoor Test Using Imocap in STA Mode
The same procedure as in protocol 3 was repeated, but this time using Imocap in STA mode, where the network support was provided by the router. The weather conditions for the experiment were the following: average temperature of 16ºC, no rain, relative humidity 72% and winds of 4 km/h. To show the Wi-Fi signal dissipation received by Imocap, a heat map has been created in Figure 7.
In Figure 8, it is possible to see the relationship between distance and the transmission strength, while in Table 5 there is a summary of the findings in this protocol.
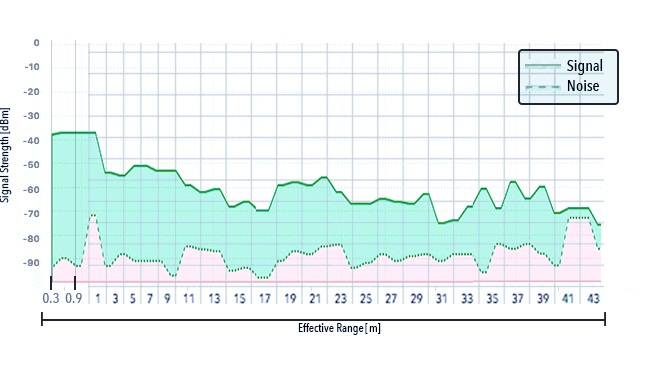
Fig. 8. Outdoor wireless signal characterization: signal strength and noise versus distance, using Imocap as Station.
Table 5. Summary of the Protocol 4: using Imocap as Station, outdoor test.
Distance [m] | Avg. Signal Strength [dBm] | Noise [%] | Avg. Trans. Speed [MB/s] | Avg. Latency [ms] | Data Quality [%] |
0.3 | -37.0 | 10 | 2.68 | 13.10 | 100 |
0.6 | -37.0 | 7 | 2.68 | 12.74 | 100 |
0.9 | -37.0 | 7 | 2.68 | 12.74 | 100 |
1 | -37.0 | 10 | 2.68 | 13.10 | 100 |
5 | -48.5 | 8 | 1.89 | 16.56 | 98 |
11 | -55.4 | 13 | 1.62 | 22.62 | 88 |
21 | -57.4 | 5 | 1.80 | 22.22 | 85 |
43 | -67.6 | 20 | 1.49 | 26.91 | 73 |
45 | -70.3 | 25 | 1.33 | 29.88 | 68 |
It can be concluded that the effective range of the biomechanical data capture system Imocap in STA mode is 44.3 m. This outcome can be associated with the noise amount perceived in the router from the Imocap system. In the worst-case scenario and at maximum distance, 68% of the information was received, with a 25% noise and interference factor, a maximum transmission speed of 1.33 MB/s as a minimum and 29.88 ms latency. In Table 6, there is a general summary of the tests carried out in protocols 3 and 4.
Table 6. Outdoor test summary.
Mode / Parameter | Imocap in AP mode | Imocap in STA mode |
Max. Signal Strength | -55 dBm | -37 dBm |
Avg. Signal Strength | -70.2 dBm | -57.0 dBm |
Max. Transmission Speed | 2.16 MB/s | 2.58 MB/s |
Avg. Transmission Speed | 1.7 MB/s | 1.8 MB/s |
Avg. Latency | 7.9 ms | 22.5 ms |
Avg. Data Quality | 10.5 % | 12% |
Effective Area | 85.8% | 84.5% |
In the outdoor case tests, the network performance created by the system in AP mode is greater, given that it has a higher percentage in the data quality, it offers coverage at a greater effective distance with lower latency and a lower noise percentage in the transmission.
IV. Discussion and Conclusions
One of the main advantages of using a capture and analysis system for biomechanical signals which have an integrated wireless communication system is to be able to offer portability to those devices that, in general, require large operating infrastructure, associated costs and qualified personnel.
Given the results obtained through the development of the test protocols, it is concluded that the transmission of biomechanical information through Wi-Fi using the TCP protocol is efficient and robust, both indoors and outdoors, even in environments of radio frequency interference. The use of this protocol is emphasized since its use allows the transmission of packages to be carried out in a controlled manner, allowing the error handling and recovery.
Specifically, for an indoor work environment, the signal maximum range when the Imocap system is in AP mode is enough to transmit data to a nearby device where a better-quality signal is obtained by it being in the same space as the receiving device. Even in the presence of multiple wireless networks, the Imocap capture system maintains its efficiency with a strong transmission connection. Under the same tests set, when the Imocap system is used in STA mode, efficiency rises by 2%, which does not mean a significant improvement. It is concluded that in any working mode while being indoors, data transmission does not differ significantly, both modes being suitable to carry out the wireless data transmission.
For an outdoor work environment, the maximum signal range when the Imocap system is in AP mode is greater than that achieved when it is in STA mode. Although the AP mode registers a lower signal intensity, the link is more stable since the noise percentage is lower than that obtained in STA mode. It should be kept in mind that the link stability in STA mode depends on the features offered by the router and, therefore, by improving the functions of this device, the results may vary.
Using the AP mode of the Imocap system, a total effective range of 45.6 m was obtained, which is 1.3 m more than the maximum range obtained in STA mode. Although there is no substantial difference in terms of effective distance or data quality, it can be affirmed that the Imocap system in AP mode is more effective in outdoor environments, since it has a signal strength of 23.1% higher than that evidenced in STA mode.
Therefore, it is recommended to use the AP mode in indoor and outdoor environments, when a fast data transmission in applications in real-time is needed since the latency is much lower than in STA mode. At the same time, the STA mode is recommended when the latency is not a critical and determining factor in the data transmission or when a previously established communication infrastructure exists. Another advantage of using the STA mode is the information centralization when it is produced by several Imocap systems that work together since a router can connect several Imocap devices in station mode and redirect the data to the final receiver.