Introduction
Last October the Royal Swedish Academy of Sciences announced the 2021 Physics Nobel Prize to be awarded to climatologists Syukuro Manabe (Princeton University, US) and Klaus Hasselmann (Max Planck Institute for Meteorology, Germany), and physicist Giorgio Parisi (University of Rome "La Sapienza", Italy). According to the Nobel Committee, the prize was given for their "groundbreaking contributions to our understanding of complex physical systems" [1]. S. Manabe and K. Hasselmann shared half-prize "for the physical modelling of Earth's climate, quantifying variability and reliability predicting global warming." The other half-prize was earned by G. Parisi "for the discovering of the interplay of disorder and fluctuations in physical systems from atomic to planetary scales."
Therefore, this year's Physics Nobel Prize focused on complex physical systems which can be characterized from large scales, such as the Earth's climate, to disordered microscopic structures. The awarded scientists were recognized due to the development of new theoretical methods that were successful to predict the behavior of complex systems.
Climate physics
S. Manabe was one of the pioneers in developing numerical models aiming at the prediction of the Earth's climate changes. In his seminal works, published about six decades ago, Manabe developed methods that are still being used at the IPCC (Intergovernmental Panel on Climate Change).
More specifically, Manabe's models allowed the development of relationships between the growth of CO2 concentration with the temperature increase in the lower atmosphere and reduction in the upper atmosphere. Manabe's works enable to evaluate the importance of reducing CO2 emissions and thus were essential to advance the understanding of the climate change in the Earth.
Klaus Hasselmann discovered how to incorporate random changes that occur in the atmospheric variables into climate prediction models. Inspired by the theory of Brownian motion, Hasselmann created a climate model that took these variations into account and allowed the evaluation of the human impact on the Earth's climate.
Spin glasses, disorder and frustration
To understand the contribution of Giorgio Parisi in the context of the Condensed Matter Physics, we initially recall his works on the study of disordered magnetic materials.
In 1972, Cannella and Mydosh observed [2] an unusual magnetic behavior in metallic alloys, in which atoms of gold and iron were randomly mixed. In such systems, the spins of the iron atoms at low concentrations and temperatures point at fixed random directions, as if they were frozen (rather slow dynamics). These magnetic alloys received the name of spin glasses (SG), in analogy with the randomness of the positions of atoms or molecules in a typical structural glass. The novelty in these systems carne from the fact that they exhibit a cusp in the magnetic susceptibility at the critical (freezing) temperature, though the net and staggered magnetizations at lower temperatures are null, indicating the absence of regular spatial magnetic order. This feature contrasts with the well-known ferromagnetic and antiferromagnetic compounds, in which the spins align parallel and anti-parallel, respectively, as well with the paramagnetic phase where spins point at random directions but display quite fast dynamics.
Disorder and magnetic frustration (i.e., the impossibility of satisfying simultaneously all spins interactions) were soon acknowledged as key ingredients to the SG phase. In 1975, Edwards and Anderson incorporated them in a model with the couplings between nearest-neighbor spins taken from a probability distribution [3]. Since the total and staggered (sublattice) magnetizations are null in the SG phase, Edwards and Anderson proposed as the SG order parameter the average correlation q = ∑ij〈SiSj〉/N between N spins S i and S j at distinct positions i and j , which is non-null (null) below (above) the freezing temperature. Though this model has succeeded in explaining most properties of SGs, it led to some physical inconsistencies, such as a negative entropy as the absolute temperature tends to zero.
Soon after this work, Sherrington and Kirkpatrick applied [4] the replica trick to evaluate powers Zn of the canonical partition function in a mean-field approach to the Ising model of infinite-range spin interactions with Gaussian distribution. Essentially, the replica trick technique works as if n replicas of the system (labelled by α = 1, 2, ...,n) with the same random coupling distribution were considered, with the mathematical limit n → 0 taken at the end of the calculation. After averaging over disorder (i.e., over the random couplings), the effective Hamiltonian displays interacting replicas. In this context, the SG order parameter is redefined as the replica overlap parameter, q α β = ∑i〈SiαSiβ〉/N. If replicas are symmetric, displaying a single q α p value independent of the replica pair (α,β), the saddle-point solution yields a SG phase either with or without external magnetic field. Nevertheless, the same problems of the Edwards-Anderson solution remained in this approach. Even worse, in 1978 de Almeida and Thouless proved [5] that the SG solution of the Sherrington-Kirkpatrick model is unstable. New ideas were thus needed at that moment.
In 1979, Parisi presented [6]-[8] a theory based on the Sherrington-Kirkpatrick model to explain the SG behavior. His main assumption was that replicas are not symmetric in the SG phase, so that the replica overlap parameter q α β can actually show a distribution of values. In the low-temperature SG phase, the distribution of q α β values present two side maxima with opposite signs, indicating that replicas are either correlated or anti-correlated. On the other hand, in the high-temperature paramagnetic phase its distribution is strongly concentrated around zero, as typical of uncorrelated (symmetric) replicas.
In the context of Parisi's theory, below the freezing temperature the free energy surface presents a multitude of local valleys and maxima that can trap distinct replicas of the SG system for relatively long times, leading to different low-temperature values of observables, slow dynamics evolution, and irreversibility properties, such as hysteresis and history-dependent measurements. This feature contrasts with the two degenerate minima in the free energy of a replica-symmetric disorder-free ferromagnet. This novel phenomenon became known as replica symmetry breaking (RSB), and Parisi's theory initiated a revolution in the study of disordered magnetic materials. Indeed, the theory has been extensively supported by many indirect comparisons with the thermodynamic, dynamics, and critical properties of various quantities, such as the magnetic susceptibility and specific heat of diverse SG materials and has also found applications in other fields of complex systems [8], such as neural networks, granular matter, and photonics, to name a few.
Random lasers and the photonic glassy phase
Random Lasers (RLs) are a class of lasers proposed by Letokhov in 1968 [9] and unambiguously demonstrated only in 1994 10. This kind of lasers differs from the conventional lasers because their operation does not require to place the active laser medium inside a conventional optical cavity. Instead, the optical feedback for laser action is provided by light scattering due to a large number of elements, atoms or ions, as well as nano- or micro-particles, with random positions within the lasing medium. Figure 1 shows a typical random laser output characterization. A recent review on RLs can be found in [11].
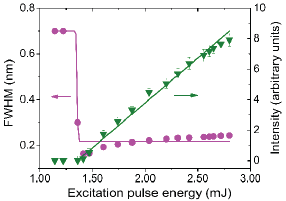
Figure 1 Emitted intensity (green) and bandwidth narrowing (magenta) as a function of the excitation pulse energy for a 3D neodymium-based RL system, showing a nice agreement of the threshold value above which the RL phase sets in (adapted from [17]).
Almost three decades after Parisi's work on disordered magnetic systems, a research group also at the University of Rome "La Sapienza" predicted theoretically a photonic analogue of the RSB glassy phase in multimode RL systems [12]. Inspired by the Hamiltonian formulation of disorder-free conventional lasers, Angelani and co-workers derived [12] a photonic RL Hamiltonian with disorder implemented in the form of Gaussian distributions of second- and fourth-order interactions among the complex amplitudes of the RL modes (the latter being related to the χ[3] nonlinear optical susceptibility). Indeed, the RL Hamiltonian is similar to that of the spherical model of continuous spin variables with second- and fourth-order Gaussian distributions, in which the spherical constraint is taken on the sum of the squared local spins (the photonic counterpart is the fixed total intensity given by the sum of the square moduli of the modes amplitudes).
The relevant fact is that this magnetic spherical Hamiltonian has been long known to provide a phase diagram displaying a low-temperature RSB SG phase [12]. In the magnetic-to-photonic analogy, the role of the absolute temperature is played by the inverse input excitation power. Similarly, the phase diagram of the RL system presents a RSB glassy phase for input powers above some threshold value, as well as a replica-symmetric prelasing phase at low input powers. In the former, the synchronous oscillation of the RL modes is frustrated, modes do not oscillate coherently in a trivial way, but are nontrivially correlated; conversely, in the latter the modes oscillate independently and incoherently.
The experimental challenge to demonstrate this theoretical prediction was fulfilled only in 2015 by Ghofraniha and co-authors [13] using a two-dimensional (2D) RL built with an organic film. In particular, since in practice the access to the phases of the lasing modes is quite difficult, the proposed replica overlap parameter was as a function of the intensity fluctuations [13],
where in the photonic context each spectrum is considered a replica emitted by the RL, i.e., a copy of its photonic signature generated under identical experimental conditions. In the above expression ∆ αí = I αi - 〈I〉 i is the intensity fluctuation of the mode i in the replica a with respect to the mode intensity (7}¿ averaged over all replicas. The normalization factor in the denominator implies q a p e [-1, 1]. Similarly to the magnetic context, the probability density function P(q) describes the distribution of replica overlap valúes q - q a ¡¡, and signáis a photonic replica-symmetric paramagnetic-like phase or a RSB glassy phase, respectively, if it presents a single maximum profile with g-values distributed around q = 0 (no RSB) or if a double peaked P(q) emerges (RSB), with saturation when the maxima P(q) occurs at the extremes q = ± 1, as demonstrated in [13].
Our group at UFPE in Recife advanced the understanding of the complex behavior of RLs in a number of directions. First, the RSB phenomenon was observed and analyzed in a quasi-lD system [14]-[16] - a random fiber laser - and in 3D RL systems [17]-[19] - both nanocrystalline powders and liquid colloids. Figures 2 and 3 illustrate the characterization of the photonic RSB SG phase in some of these systems from the analysis of the P(q) distribution profiles and the linewidth and emitted intensity as a function of the input power. The works in Recife constitute important demonstrations of photonic phase transitions that verify the RSB SG theory. Indeed, the arricies [16], [17], and [18] were referred in the Report of the Nobel Committee [1] as references [34], [33] and [74], respectively.
We have also contributed [15],[17],[20],[21] to the understanding of the nontrivial long-tailed Lévy-like distributions of output intensities in RLs (see Fig. 3). Lévy statistics is typical of systems exhibiting strong fluctuations, which are generally not accounted for by considering conventional statistical physics models based on weak Brownian fluctuations dynamics and the central limit theorem. In connection with the Lévy statistics, we have also investigated the emergence of extreme events of emitted intensity valúes in a random fiber laser [22].
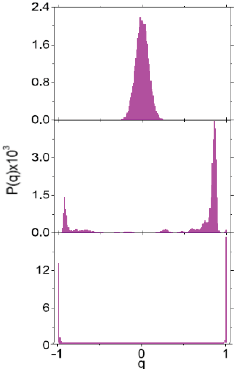
Figure 2 Distribution P(q) of valúes of the replica overlap parameter q of a 3D neodymium-based RL system for excitation pulse energies below (top panel), at (middle), and slightly above (bottom) the threshold. A replica-symmetric prelasing phase is associated with P(q) centered around zero, whereas in the photonic RSB glassy phase P(q) displays two side máxima (adaptedfrom [17]).
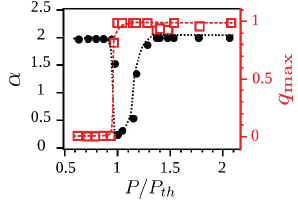
Figure 3 Valúes of q max where P(q) is máximum and Lévy index a as a function of the excitation pulse energy relative to the threshold valué, P/P t h, for a ID erbium-based random fiber laser. Below the threshold, P/P t h < 1, one notices that q max ~ 0 and a = 2, characterizing the replica-symmetric prelasing phase with Gaussian distribution of weakly fluctuating emitted intensities. For P/Pa, > 1 a photonic RSB SG phase sets in as qmax ~ 1, displaying strongly fluctuating RL intensities with Lévy distribution of index 0 < a< 2 (adaptedfrom [15]).
More recently, we have also provided the first observation of a Floquet phase in a photonic system by considering a nearly periodic power source and analyzing the time-dependent counterpart of the distribution of the replica overlap parameter [23]. Moreover, in another direction we have analyzed the intensity fluctuations between consecutive output spectra and found out a turbulent-like emission regime in which their long-tailed distribution has stretched exponential form [23]. These results were obtained in the theoretical framework of a statistics superposition model where the background distribution arises from a hierarchical system of stochastic differential equations with multiple scales.
Conclusions
Complex systems are ubiquitous in Nature, and the 2021 Nobel Prize of Physics has acknowledged the contribution of Syukuro Manabe, Klaus Hasselmann, and Giorgio Parisi to advance their understanding. The importance of the contributions by Manabe and Hasselmann can be hardly overstated since climate issues are nowadays a global priority. Further, the work of Parisi has ignited a revolution in the statistical physics approach to complex systems, starting from the magnetic spin glasses but going much beyond to reach areas such as neural networks and photonics. Our group at UFPE in Recife has helped to pursue the idea of using random lasers as a statistical platform to study the various challenging aspects of complex systems. Though much progress has been achieved so far, a flourishing path can be foreseen to the future theoretical and experimental investigation of complex systems.