Services on Demand
Journal
Article
Indicators
-
Cited by SciELO
-
Access statistics
Related links
-
Cited by Google
-
Similars in SciELO
-
Similars in Google
Share
CT&F - Ciencia, Tecnología y Futuro
Print version ISSN 0122-5383On-line version ISSN 2382-4581
C.T.F Cienc. Tecnol. Futuro vol.2 no.2 Bucaramanga Jan./Dec. 2001
ABSTRACT
Over the past years, environmental regulations have driven a lot of effort for the development of new technologies for the upgrading of fossil fuels. Biotechnology offers an alternative way to process fossil fuels by means of a biodesulfurization technolgy where the production of the biocatalyst is one of the key topics. Traditionally, the production is carried out in batch culture where the maximum cellular concentration is restricted by inherent limitations of the culture type and the microorganism growth rate. This work adresses the production of two desulfurizing microorganisms: Rhodococcus erythropolis IGTS8 and Gordona rubropertinctus ICP172 using fed-batch culture. Fed-batch cultures were conducted in a 12 L fermentor using ICP4 medium containing glucose and DMSO as carbon and sulfur sources. As a result, cell concentration was increased 1.5 and 3 times with fed-batch cultures using constant and exponential flow respectively, achieving a maximum cell concentration of 7.3 g DCW/L of biocatalyst IGTS8 and 12.85 gDCW/L of the new biocatalyst ICP172. Both biocatalysts presented biodesulfurization activity in a spiked matrix (DBT/HXD) and in Diesel matrix with the detection of 2-HBP, which is the end-product of DBT degradation pathway.
Keywords: biocatalyst, biodesulfurization, high density cultures, fed-batch cultures.
RESUMEN
El mercado de combustibles exige el desarrollo de nuevas tecnologías para la remoción de azufre orgánico. Dentro de las alternativas en desarrollo, la Biodesulfurización de Hidrocarburos (BDS) resulta promisoria por su selectividad y posible competitividad económica. La producción del biocatalizador es uno de los puntos claves en el desarrollo de la tecnología de BDS. Tradicionalmente la producción se realiza mediante cultivos lote en donde la concentración celular máxima es restringida por limitaciones inherentes al tipo de cultivo y por la velocidad de crecimiento del biocatalizador. En este trabajo se logró incrementar la concentración celular del biocatalizador comercial IGTS-8 y de la cepa nativa ICP172, utilizando cultivos por lote alimentado con 2 diferentes estrategias de alimentación. Los incrementos celulares con respecto al cultivo lote fueron de 1.5 veces y 3 veces en cultivos lote alimentado con flujo constante y exponencial respectivamente, logrando concentraciones celulares máximas de 7.3 gr/L con el biocatalizador IGTS-8 y 12.85 con el nuevo biocatalizador ICP172. Los biocatalizadores obtenidos presentaron actividad biodesufurizadora tanto en matriz ideal (DBT/HXD) como en matriz real (Diesel). El incremento en la productividad del biocatalizador significa un importante aporte desde el punto de vista de desarrollo del proceso de Biodesulfurización, considerando las altas concentraciones que se requieren para la reacción de contacto con la matriz a desulfurizar.
Palabras clave: biocatalizador, biodesulfurization, culturas de alta densidad, cultivos lote alimentado.
INTRODUCTION
Industrial development has resulted in environmental damage noticed in global heating, acid rain, and the ozone layer's destruction. To protect the environment and grant the well-being for future generations, governments have set laws for controlling atmospheric emissions, liquid resources, and solid waste coming from industrial activities.
In the petroleum industry there are laws to decrease atmospheric emissions in refining processes, mainly carbon dioxide which affects the global heating or "greenhouse effect", and nitrogen and sulfur oxide's emissions that contribute to acid rain. For these reasons the U.S. Environmental Protection Agency (EPA) and other worldwide regulating agencies, have restricted the amount of sulfur in diesel and gasoline.
Traditional processes for the removal of sulfur like hydrodesulfurization (HDS) have several technical and economical challenges to overcome in meeting these low sulfur concentrations in diesel and gasoline. HDS plants or units are not only expensive to construct but also to operate because of the use of gassy nitrogen at high pressures (150-3,000 lb/in2) and temperatures 563K - 728K (290°C-455°C). In addition, this chemical process is limited in removing certain molecules from the petroleum, especially polyaromatic compounds found in heavy fractions like dibenzothiophene (DBT) and related compounds.
Biotechnological processes to remove sulfur from petroleum have been developed (Monticello D.J., 1998). There are several known microorganisms capable of desulfurizing DBT without degrading the carbon and calorific value of these compounds. Because of its specificity and low costs, biodesulfurization is a promising alternative to produce low-sulfur-containing diesel and gasoline.
The Empresa Colombiana de Petróleos - Instituto Colombiano del Petróleo (ECOPETROL-ICP) is hard- working to systematically implement a biodesulfurization technology by a coordinated strategy addressing both process and biocatalyst development. The development of a petroleum biodesulfurization process requires multiple topics of investigation: the design of the biocatalyst's production process, the development of a two phase reactor system, the isolation and genetic modifications for new biocatalysts with improved features like higher catalytic activity, shorter generation time and tolerance to the process' conditions which are high temperature and high solvent concentration.
Considering that a biodesulfurization process requires near 20-40 gr/L of biocatalyst and the process must be achieved in a continuous form and at industrial scale, the biocatalyst's production rate is an important fact in the process' development.
Batch culture has been traditionally employed to produce the biocatalyst needed for the desulfurizing process both for growing (Lee et al., 1995) and steady (Folsom et al., 1997,Oshiro 1996) cell schemes. However, only a few groups have analyzed other cultivation techniques. Honda et al., 1997, developed a high density culture strategy with strain IGTS-8 obtaining cellular concentrations of 30 gr/Lin a fed-batch culture, bymeans of an indirect system that uses the pH value to estimate the concentration of the acetic acid. The pH was controlled by adding pulses of a concentrated solution of acetic-acetate ammonium acid. Moreover, Wang et al., 1996, developed a continued culture system to determine the kinetic parameters of culture for the strain Rhodococcus erythropolis N1-36, accomplishing a maximum growing rate (µmax) of 0.235 h-1 and a cellular concentration of 4.3 g. biomass/L. In the same year (1996), Wang and Krawiec published results for batch and fed-batch cultures kept during 200 hr (eight days), accomplishing cellular concentrations of 1.3 gr/L for batch culture and 4.3 gr/L for fed-batch culture. However none of the reported cases of fed-batch cultivation of desulfurization-competent Rhodococcus sp. cultures specifies the feeding profile. Other authors like Oshiro et al., 1996, and Izumi et al., 1996, achieved a maximum cellular concentration of three units at (OD660).
The published literature does not show a clearly helpful strategy to increase biocatalyst production for use in a desulfurizing process. Hence, it is relevant to evaluate different culture strategies to achieve high cell density cultures (HCDC). HCDC have been reported to achieve cellular concentrations as high as 100 g/L. Initially, HCDC techniques were employed to obtain ethanol and cellular protein from yeast and has also been applied to Escherichia coli cultures (Reisenberg and Guthke, 1999). The maximum cellular concentration depends on the microorganism and the employed strategy. Wandrey et al., 1986 cited in Reisenberg and Guthke, 1999 achieved cellular concentrations of 95 g/L in Corynebacterium glutamicum cultures and 80 g/L in Brevibacterium flavum cultures. Moreover, Park et al. 1992, achieved concentrations of 185 g/L in Bacillus subtilis cultures. Some species of microorganisms are more difficult to grow, nevertheless, the use of HCDC techniques achieved concentrations of 30-40 gr/L in Chromobacterium violaceum and Pseudomonas oleovorans (Steinbuchel and Schmack, 1995, Preustin et al., 1995, Preustin et al., 1993). Moreover, Honda et al., in 1998 achieved a cellular concentration of 30 g biomass dry weight/L, using HCDC techniques with Rhodococcus rhodochorus (IGTS-8) in 28 h, which is equivalent to a volumetric productivity of 1.17 g biomass dry weight/(L-h).
The fed-batch culture is the most commonly used strategy for HCDC, this is a semi-continuous system where the nutrients are supplied continuously or semicontinuously to maintain limiting concentrations of one or several substrates and avoid the accumulation of inhibitory metabolites due to substrate surplus. Afeeding strategy could be designed based on the knowledge of the concentration of a growth limiting substrate or based on the system's kinetic parameters. Some authors have classified fed-batch cultures according to the feeding strategy. There are two cases: fed-batch culture at variable volume and fed-batch culture at pseudo-constant volume. In the first case, the culture is started with a percentage of the reaction volume and progresses with a constant or variable flow. In the second case, the initial volume is almost the reaction volume and is fed with a solution of limiting substrate, this strategy usually has a control system that allows feeding by means of a signal.
This work evaluated two fed-batch culture strategies with variable volume, to increase the production of Rhodococcus erythropolis IGTS8 and a new desulfurizing biocatalyst Gordona rubropertinctus ICP172.
MATERIALS AND METHODS
Microorganisms and culture conditions
Tests were made using Rhodococcus erythropolis IGTS-8 (ATCC 53968) and a native desulfurizing strain ICP172 (Gordona rubropertinctus), isolated previously from oily sludges. Strains were maintained in LB agar at 277K (4°C). Preinoculum for the fed-batch cultures were prepared firstly in LB broth for 36 hr at 305K (32°C), then a dilution 1:10 was used as inoculum of 2L flasks with aworking volume of 600 mL of ICP4 culture media. Cultures were kept during 36 hr in orbital agitator at 140 rpm and 305K (32°C). ICP4 culture media contained per liter of deionized water: Glucose 12.4 g, Na2HPO4 3.74 g, KH2PO4 1.68 g, NH4Cl 2.84 g, MgCl2· 6H2O0.146 g, FeCl3· 6H2O0.02 g, CaCl3·2H2O 0.112 g, Dimethyl sulfoxide DMSO (Sigma) 20mg. The sulfur source for the growth of both biocatalysts was DMSO while Diesel (3,500 ppm of total sulfur) and DBT in hexadecane (1,040 ppm sulfur) were used in the biodesulfurization tests.
Development of fed batch cultures at constant and exponential flow
Fermentation processes were carried out in a New Brunswick Bioflo 3,000 with a working volume of 12 L, instrumented with temperature control, monitoring of dissolved oxygen and air flow. Initially the microorganism is grown in batch followed by culture media feeding with an exponential flow determined by this equation: F=Vo*D*exp(µ*t), where µ represents the microorganism growth rate, D is the dilution rate and Vo is the initial volume. Feeding profile was based on the microorganism growth rate, feeding was suspended whenever the growth slope decreased and was reinitiated when cellular concentration started to increase. Cellular concentration was determined by means of the absorbance at 600 nm in a Hewllet Packard spectrophotometer, biomass dry-weight was calculated based on a previously performed dry-weight standard curve.
Desulfurization reactions
Desulfurizing activity of the biocatalysts were evaluated by means of resting cells' tests standarized at ICP's Biotechnology Laboratory as follows: biomass (biocatalyst) produced by fed-batch culturewas gathered by centrifugation in aseptic conditions and washed three times with phosphate buffer 0.01 M followed by an activation stage where biomass is placed in ICP4 culture media with DBT. Biomass was washed with phosphate buffer 0.01 M and the final pellet was preserved at 277K (4°C) during eight hour according to the adopted protocol in the laboratory.
Biodesulfurization reactions were performed in a Biostat BRAUN D reactor with a working capacity of 2 L. Activated biomass was resuspended in 750 mL of phosphate buffer 0.15 M with 2% of glucose as the carbon source, the suspension was kept during six hours under controlled conditions of aeration 0.3-0.45 vvm, agitation 300 rpm and temperature 303K (30°C), this was called the exhaustion stage. Finally, 250 mL of Diesel (3500 ppm of total sulfur) were added keeping a volumetric water-to-oil ratio of 3:1 under the same conditions. Sampleswere taken at regular time intervals to analyze total sulfur concentration. Samples were centrifuged at 3,180 g during five minutes to separate biocatalyst and aqueous phase from the organic phase.
Analitic determinations
Glucose concentration was determined according to Bernfeld (1955). Total sulfur concentration was quantified by means of gas chromatography attached to a photometric flame detector. A PB-1 fused silica capillary column (25mx 0.32mmx4mm) was used. The injector temperature was 563K (290°C). Dimethylsulfoxide (DMSO) was determined by means of liquid chromatography (HPLC). DBT and 2-HBP were quantified by means of a gas chromatography with a flame ionization's detector (GC/FID). The analysis for DBT and 2-HBP were performed on a Hewlett Packcard 6890 gas chromatograph equipped with a flame ionization detector (FID) and on HP 7673 auto-sampler. A HP-1 fused silica capillary column (50mx 0.2mmx 0.5mm) was used. The carrier gas was helium (1.5ml/min). The injector and detector temperature were 523K (250°C) and 553K (280 °C), respectively. Temperature program was: 383K (110°C) ramp at 298K/min (25°C/min) to 493K (220°C), hold 1min; 493K (220°C) ramp at 280K/min (7°C/min) to 583K (310°C), hold 5 min. A1ml aliquot was injected in the split mode (10:1).
RESULTS AND DISCUSSION
The first strategy analyzed for the production of desulfurizing biocatalysts was the one recomended by Honda et al., 1997, pH-stat, based on the feeding of the carbon source in response to the pH value. The maximum cell yield with this strategy was 5 gr/L of biocatalyst IGTS8 in 70 hr, compared to the results obtained by Honda et al., of 30 g/L. In this case, there was also an accumulation of the carbon source to limits where it turned inhibitory for the cells. Since this fermentation process is highly sensitive and there was no complete automated control of the parameters at the laboratory (data not shown), two other open loop culture strategies were analysed: constant and exponential feeding profiles.
Fed-batch culture at constant flow
The design of a fed batch culture for the production of biocatalyst IGTS8 included an initial batch culture (3 L) followed by a feeding stage with constant flow profile F(t), equal to 2 mL/min. This feeding profile was an initial attempt of adjusting the volume's increasing slope to the microorganism exponential growth slope previously determined. The feeding stage was mantained continuously up to 80% of the total reactor's volume (12 L). A partial discharge of 9 L was performed holding 3 L in the reactor as inoculum for a second fedbatch culture under the same feeding scheme. The culture's behavior during the first phase is presented in Figure 1. Around 60 hr there was a reduction in the growing slope of the microorganism where the feeding profile was started. During the first hours the cellular concentration did not increase because of a dilution effect, nevertheless, after an adaptation period the cellular concentration started to increase up to a density of 4.5 g/L. This concentration is 1.5 times higher than the batch culture of the same microorganism developed by our group with ICP4 media (data not shown) and the same concentration obtained by Wang and Krawieck (1996), using a similar cultivation strategy. Figure 1 also presents the consumption kinetics for the carbon (glucose) and sulfur (DMSO) sources. The carbon source is consumed at a rate of 65 mg/L.h during the feeding phase, without total exhaustion. The reduction in the growth rate of the microorganism after 127 hrmight be due to differents factors: a substrate limitation different to the carbon and the sulfur sources, inhibiting metabolites accumulated in the culture and/or insufficient feeding flow to maintain the growing rate. These conditions may be overcomed by the identification of inhibitory compounds, the limitation of nutrients responsible for their production and their removal by a semicontinuous culture design.
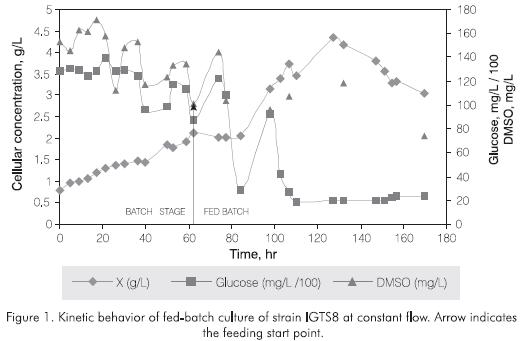
A second feeding phase was performed under the same conditions after a partial discharge at 160 hr - not shown in the figure-. This second phase didn't present
an important increase in the cellular concentration, consumption kinetics for the sulfur and carbon sources reveled that there were no limits in any of these nutrients with remaining concentrations of 100 ppm of DMSO and 80 ppm of Glucose.
A growth limitation in this kind of fermentation strategy was also observed by Kellerhals et al., 1999 in the Polihydroxyalkanoate's production with Pseudomonas oleovorans, around 35 hr of culture. The fact that during constant flow, an increase in cellular concentration would not be proportional to the nutrients's supply, could cause substrate's limitation and hence a reduction in the microorganism growth rate. This can also be seen for IGTS8 in Figure 2 where at the beginning of the feeding strategy both, the growth slope and the nutrients supplywere directly related, after 100 hr of culture there was a limitation in nutrients supply.
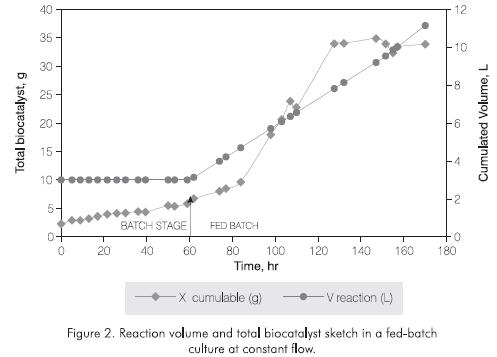
Figure 2 shows the reaction volume sketch according to the constant feeding flow. An increase in the biocatalyst weight in the fed-batch culture stage reflects the benefits of the fermentation strategy.
Fed-batch culture at exponential flow
Considering the exponential growth of microbial cultures, the substrate's requirements can be satisfied by means of a feeding strategy where the volume is being incremented according to equation (2).
Where D is the dilution rate and Vo is the initial volume. If F is given by:
Deriving 1 in time and replacing 2 we have got:
Where µ: Growth rate.
In fed-batch cultures at exponential flow, the dilution rate is constant, hence it can be established a dilution rate equivalent to the culture's maximum growing rate and operate it under this condition, this is D = µ.
In this case, as in fed-batch culture at constant flow, the culture was initially performed in batch (3L) followed by a feeding phase according to the profile defined by equation 3 for a dilution rate (D) of 0.03 h-1 which was the maximum calculated in ICP4 culture media for strain IGTS-8. It seems like the dilution ratewas overestimated since no increment in the cellular concentration was observed (Figure 3 around 75 hr of culture). It was then necessary to suspend the feeding until the growth was re-established between 100 and 190 hours of cultivation. Subsecuently, the feeding profile was recalculated with a dilution rate of D 0.01 h-1 until total volume reached 12L. Under these conditions it was possible to increase the cellular concentration up to 7.5 gr/L as shown in Figure 3. Glucose consumption was higher than in fed-batch culture with constant flow where glucose might have been a limiting factor while DMSO (sulfur source) was not exhausted.
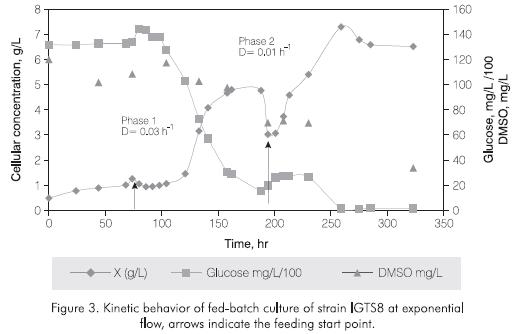
Figure 4 shows the volumetric profile during the batch and exponential feeding profile for the production of strain IGTS8. Their related slopes were D=0.03 h-1 for the first feeding phase and D=0.01 h-1 for the second feeding phase. This strategy resulted in a cellular concentration of 7.3 g/L in aproximately 200 hr of culture. This concentration is three times the obtained in batch culture and 1.7 times the reported by Wang and Krawiec (1996) who worked in 1.5 L bioreactors.
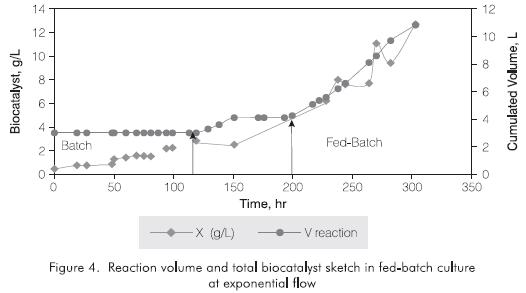
The same feeding strategy was evaluated for the native strain ICP172 which presents similar growing conditions as IGTS8 strain in ICP4 medium. Strain ICP172 presented a growth rate (µ) of 0.015 h-1 in batch culture, for instance D= 0 · 015 h-1. Figure 5 shows the fed-batch culture with exponential flow for strain ICP172. Under this feeding profile and for about 150 hr the cellular concentration did not increase, culture media feeding was put off and the culture was mantained in batch for 193 hr when cellular concentration started to increase. F was recalculated in equation 3 with D = µ = 0.01 h-1 and Vo= 4L. The culture medium was fed until a total volume of 12L. During this second feeding phase it was possible to mantain the culture in exponential growth with µ=0.012 h-1 and reaching a cellular concentration of 12.85 g/L. This cell yields are closely related to the ones reported by Folsom et al., 1999, for IGTS8.
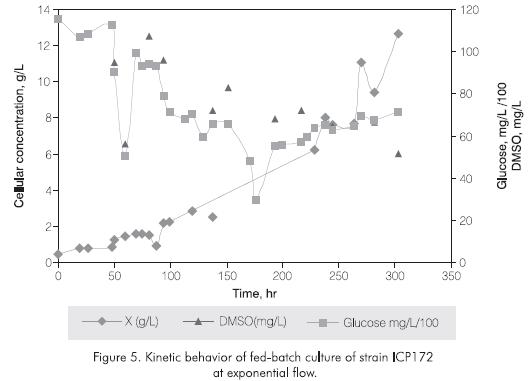
Figure 6 shows the total production of ICP172 biocatalyst and the feeding profiles analysed. With this feedind profile it was possible to dose the nutrients according to ICP172 growth rate (190 - 300 hr).
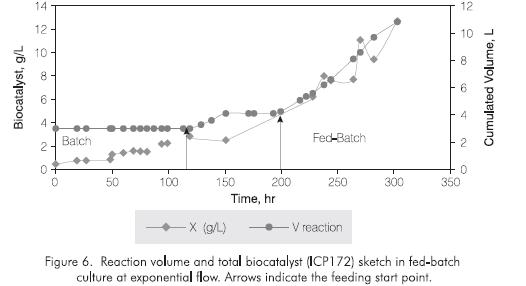
Desulfurization reactions
In every case biocatalyst IGTS8 and ICP172 produced by fed-batch culture were catalytically active in the biodesulfurizing test swith DBT in hexadecane and among Diesel. All the desulfurizing tests were analysed for the detection of 2-hydroxybiphenyl the final metabolite in the desulfurization route 4S (data not shown).
CONCLUSIONS
-
Fed-batch culture is an alternative to increase the production of biocatalysts with desulfurizing activity, despite the low growth rate of these microorganisms.
-
Exponentially fed-batch culture was analyzed for a new desulfurizing biocatalyst, G. rubropertinctus ICP172. Cellular yields were higher due to the possibility of dosing the nutrients according to particular microorganism's growth needs.
-
It was possible to increase the fermentation scale from 500 ml to 12 liters and improve cellular concentrations up to 12.85 gDCW/L with a feeding strategy that doesn't require total automated equipment.
-
Biomass productivity can be increased with additional improvements like: detection and remotion of inhibiting metabolites, identification of limiting substrates and dosing by means of automated control systems.
-
Fed-batch culture strategy can be improved working at different dilution rates and developing control algorithms that allow to operate the feeding flow according to physical and chemical changes in the cultures like dissolved oxygen, pH and turbidness peaks.
-
Biocatalysts, IGTS8 and ICP172 cultivated by fed-batch culture were active in desulfurization experiments in DBT/HXD and Diesel fuel with the production of 2-HBP.
ACKNOWLEDGMENTS
The authors express thankfulness to the researchers of hydrocarbon biodesulfurization project at ICP, specially to the Bacteriologist Adriana Salcedo, the Chemist Alix Patricia Jaimes and the Chemical Technician Alexis Rodriguez.
REFERENCES
Bernfeld, R, 1955. "Amylases". In: Methods of Enzymology, Colowick, A. and Kaplan, B., New York, Academic Press, 1:149-150. [ Links ]
Folsom, B.R., Schieche, D.R., Dgrazia, P.M., Werner, J. and Palmer, S.K., 1999. "Microbial desulfurization of alkylated dibenzothiophenes from a hydrodesulfurized middle distillate by rhodococcus erythropolis 1-19". Appl. and Environ. Microbiol., 65 (11): 4967-4972. [ Links ]
Honda, H., Sugiyama, H., Saito, I. and Kobayashi, T., 1998. "High cell density culture of rhodococcus rhodochrous by ph-stat feeding and dibenzothiophene degradation". J. of Fermen. and Bioeng., 85 (3): 334 - 338. [ Links ]
Lee, M.K., Senius, J.D. and Grossman, M.J., 1995. "Sulfurspecific microbial desulfurization of sterically hindered analogs of dibenzothiophene". Appl. and Environ. Microbiol, 61 (12): 4362 - 4366. [ Links ]
Monticello, D.J., 1998. "Riding the fossil fuel biodesulfurization wave". Chemtech, 28 (7): 38-45. [ Links ]
Ohshiro, T., Hirata, T. Y. and Izumi, Y, 1996. "Desulfurization of dibenzothiophene derivates by whole cells of rhodococcus erythropolis H-2". FEMS Microbiol. Letters, 142: 65 -70. [ Links ]
Park, Y, Kai, K., Iijima, S. and Kobayashi, T., 1992. "Enhanced b-galactosidase production by high cell-density culture of recombinant bacillus subtilis with glucose concentration control". Biotechnol. and Bioeng., 40 : 686-696. [ Links ]
Preusting, H., Van Houten, R., Hoefs, A., Van Langenberghe, E., Favre-bulle, O. and Witholt, B., 1993. "High cell density cultivation of pseudomonas oleovorans: Growth and production of poly (3-hydroxyalkanoates) in two-liquid phase batch and fed-batch systems". Biotechnol. and Bioeng, 41: 550-556. [ Links ]
Riesenberg, D. and Guthke, R., 1999. "High cell density cultivation of microorganisms". Appl. Microbiol. Biotechnol. 51:422-430. [ Links ]
Steinbüchel, A. and Schmack, G, 1995. "Large-scale production of poly (3hydroxyvaleric acid) by fermentation of chromobacterium violaceum, processing and characterization of the homopolyester". J. Environ. Polym. Degrad., 3:243-258. [ Links ]
Wang, P. and Krawiec, S., 1996. "Kinetic analyses of desulfurization of dibenzothiophene by rhodococcus erythropolis in batch and fed-batch cultures". Appl. and Environ. Microbiol., 62 (5): 1670 -1675. [ Links ]
Wang, P. Humphrey, A.E. and Krawiec, S., 1996. "Kinetic analyses of desulfurization of dibenzothiophene by rhodococcus erythropolis in continuous cultures". Appl. and Environ. Microbiol, 62 (8): 3066-3068. [ Links ]