1. INTRODUCTION
FAME (fatty acid methyl esters) represents a renewable, non-toxic, clean combustion chemical alternative with the potential to become a supplementary or substitute source for traditionally used fuels [1] One of the disadvantages of FAME is the costs associated with the use of high quality raw materials [2], [3], In recent years, part of the research related to the production of FAME has focused on obtaining a reusable catalyst and offering environmental advantages over the traditional catalysts: lipases. One of the greatest advantages of this biocatalyst is the conversion of low quality raw materials (high free fatty acid content >0.5%), which can reduce FAME costs associated with the refining of raw materials [4]-[6].
This research integrated the aforementioned studies through a heterogeneous enzymatic kinetic analysis for FAME production A simultaneous hydroesterification and alcoholysis mechanism previously reported in the literature, was used to take into account the products of the intermediate reactions and the limitations by mass transfer when using the enzymes in immobilized form Sulaiman [2],[7],[8], Experimental validation of the proposed model was performed by testing an immobilized lipase from Thermomyces lanuginosus.
2. THEORICAL FRAMEWORK
Lipases are versatile enzymes widely used in different types of reactions. However, the use of lipases in their native form is often hampered by several limitations, such as high costs, low operational stability in unusual mediums such as organic media and difficulties in recovery and reuse [9],[10]. These drawbacks could be overcome through the immobilization of lipases [11]. Different procedures have been used to immobilize lipases; however, the physical adsorption on hydrophobic supports has been the most widely used because it is a simple, gentle and cheap protocol to prepare active and robust biocataiysts [10].
Mesoporous silicates are promising candidates for lipase adsorption with respect to the requirements of enzyme carriers, i.e. large surface area, narrow pore size distribution, well-defined pore geometry, their thermal and mechanical stability and toxicological safety. Furthermore, the surface of silica supports can be chemically modified with various functional groups [9],[10], [12], Depending on the type of functional group on the surface of the support and the degree of hydrophobicity, different types of interactions between the enzyme and the support can be promoted. Lipases possess a catalytic mechanism known as interfacial activation, where a Lid that covers the active site in most lipases is modulated from a closed form (inactive enzyme) to an open form (active enzyme) in the presence of hydrophobic interfaces [10]-[13], Thus, an adequate immobilization protocol should promote hydrophobic interactions between lipase and support. A successful method for immobilizing lipases on Octyl-silica supports has been reported by different authors [10]-[12],[14],[15], This method acquires a strong hydrophobic character that can promote the hydrophobic interactions and interfacial activation of lipases. Different authors have independently reported the mass transfer analysis of immobilized enzymes and enzymatic kinetics using the enzyme free form for FAME production [2],[8],[16]-[20]. Based on this, the described method was used in this study to immobilize a process for the lipase Thermomyces Lanuginosus.
3. EXPERIMENTAL DEVELOPMENT
MATERIALS
HPLC grade methanol (99.90%) of Merck brand, enzymatic solution (lipase from Thermomyces lanuginosus, specific hydrolysis activity of olive oil measured at 3405.33 U/g ± 244.00 U/g and protein content measured at 15 mg/mL using the Bradford method) deionized water and refined, bleached, and deodorized palm oil (RBDPO) were used. The RBDPO had an acidity index of 0.16 mg KOH/ g Oil (using ASTM D664 standard method), density of 0.896 g/ml (34°C) and viscosity of 44.68 cP (34°C). Table 1 shows the fatty acid profile of the refined, bleached, and deodorized palm oil,
PROCESS CONDITIONS
To find the best process conditions with free enzyme, a 3 factor central composite design (CCD) was used in two levels, based on the methodology described by Castro-Posada [21]. Due to the thermostability restrictions and possible inhibition of the enzyme by high methanol concentrations in the medium, the higher temperature and substrates molar ratio were set at 38 °C and 6, respectively The higher amount of enzyme used was established in accordance with the literature on similar enzymatic liquid formulations [22]-[24], The other variables were set in constant values according to recommendations found in the studies [25],[26] and available information on the commercial product Eversa Transform [24], The presence of solvents in the reactive medium entails the addition of more post-process separation stages, therefore no solvents were used in this study. The factors that were set at fixed values were the amount of water (2 % of the oil weight) and the stirring (200 ±1 rpm) Based on the development of the CCD experimental tests and adjusting the data to a second-degree polynomial using response surface methodology (RSM), the optimum conditions for the FAME production process were proposed as follows:
Percentage of enzyme = 1.10 %
Temperature = 34.0°C±0.1 °C
Methanol: oil molar ratio = 4.10:1
These were the experimental conditions used for validating the kinetic model.
Table 2 shows the density and viscosity of different substances used in the construction of the kinetic model. These properties were obtained from Aspen Plus® version 8.6, for a temperature of 34 ° C.
IMMOBILIZATION
The immobilization support was functionalized with Octyl-groups in accordance with the methodology described by Lima et al [11]., using a mesoporous support [11]. Then, the immobilization process of the enzyme was developed as per the literature [11], [27] the immobilization process consisted of preparing a suspension containing the enzymatic solution, previously prepared in a sodium phosphate buffer solution, with a provision of 100 mg protein/g of support. The suspension was stirred for 24 hours at 25°C. Finally, the immobilized support was filtered and washed with deionized water [11].
The characterization of the immobilized support is explained in detail in the work by Castro-Posada [21]. The resulting immobilized support had a load of 56.5 mg of immobilized protein/g and approximate average hydrolytic activity of 347 U/g of immobilized support [21].
EXPERIMENTAL DATA
The experimental data that served as input information for preparation of the kinetic model were obtained through the assembly of independent systems in Erlenmeyers, fed with the same amount of oil, methanol, water and enzyme. The temperature and stirring speed of every sample was controlled using a thermostatic bath. The methanol supply was performed at different times (0,3 and 6 hours) to avoid the inhibition of the enzyme by high methanol concentration in the reactive medium [25],[28], In each Erlenmeyer, the reaction was stopped by thermal inhibition at 100 SC in accordance with a specified reaction time (2, 4, 6, 8, 9,10,12,18 and 24 hours) [23].
In this study, the same amount of protein was used in free enzyme and immobilized enzyme assays, respectively. The Bradford method was used to determine protein content in enzymatic solutions, while thermogravimetric analysis was used to determine protein content in the enzymatic immobilized [21], About 50 grams of oil were used in each experimental test. Therefore, the amount of enzyme solution used was 0.550g, containing 7.270 mg protein. On other hand, the protein content of the immobilized support was 56.5mg protein / g-immobilized. Thus, in each experimental test applying immobilized enzyme, an amount of 0.128 g of immobilized support was used
SAMPLE ANALYSIS
The quantification of monoglycerides, diglycerides, triglycerides and FAME was performed by gas chromatography on an Agilent 7890A Chromatograph with a flame ionization detector-FID and helium as carrier gas, using the ASTM D6584 standard as a reference. About 30 mg of every sample were diluted with a solution of pyridine, which had two standard substances for quantifying the acylglycerides percentage (butanetriol and tricapirine). An external calibration curve with 4 standard substances (oleic, linoleic, stearic and palmitic acid methyl esters) was used for quantifying FAME percentage. 1μL of the samples was injected into the Chromatograph, which was equipped with a capillary column (DB-5ht 30m x 0.25mm x0.10|im) operating under the following temperature program: 50 °C, isotherm for 1 min; ramp at 30 °C/min to 380 °C and isotherm for 10 minutes, A flame ionization detector operating at 380°C was used. Helium was used as the carrier gas at 3 ml/min,
The fatty acids concentration was obtained by titrating the sample with a known concentration of KOH solution, using the ASTM D664 standard as a reference.
ADJUSTMENT OF KINETIC MODEL PARAMETERS
This was done by using the MATLAB® R2014b program with an optimization subroutine. The method used to adjust the kinetic model parameters consisted of minimizing the sum of the squares of the relative difference between the values of the experimental concentrations and the model for triglycerides, diglycerides monoglycerides, free fatty acids and methyl esters, according to the following equation [7], [29]:
Where C ij-simulated represents the concentration of species i product of the simulation at a time j, while C ij-experimental represents the concentration of species i experimentally obtained for the same time j.
KINETIC MODEL DEVELOPMENT
The kinetic model developed comprised differential and globalized parameters.
LIMITATIONS BY MASS TRANSFER WITH ENZYME IN FREE FORM
To determine the limiting step of the enzyme-catalyzed process in free form, the mass transfer rate and reaction rate were compared.
The presence of two immiscible liquid phases, an aqueous phase and an oleic phase were considered. The aqueous phase consisted of water and propylene glycol (preservative agent for the enzymatic solution) [22], while the oleic phase consisted of oil and methanol. The enzyme was deemed as being within the oleic phase and the species to be transferred was the water.
To calculate the water transfer rate from the aqueous phase to the oleic phase, a mass transfer model was used between two immiscible liquid phases in a series [30],[31]. Thus, the following expression was obtained to calculate the mass transfer rate from the aqueous phase to the oleic phase:
It is assumed that the aqueous phase was grouped as a sphere immersed within the oleic phase and that there was friction generated by the relative motion between the two. Thus, the Sherwood number was calculated as [31]:
Assuming that the volumes of the miscible substances in the water are additives, when the water and the propylene glycol are combined, they may occupy a volume of aqueous phase V ac . Then, based on the equation for the calculation of volume of a sphere, it is possible to predict the diameter of the immersed sphere:
For purposes of calculating the Reynolds number, the aqueous phase was a free-moving sphere inside a stirred vessel. The Archimedes number (Ar) was calculated for the aqueous phase and then the Reynolds number (Re) was generated based on the value taken from the Archimedes number [32],[33].
For Ar<36:
For 36<Ar<8x104
For 8x104<Ar<3x109
Subsequently, the Schmidt number was calculated (Doran, 2013)
Finally, knowing the Sherwood number, the value of K L1 can be calculated as follows:
For the estimation of the diffusion coefficients with very diluted liquid mixtures, the Wilke and Chang correlation was used [34]:
In order to calculate the real diffusivity coefficients, simple expressions can be used as combinations of the coefficients of infinite diffusion for each single species. One of the expressions for binary blends is [34]:
Where x 1 and x 2 are the mass fractions of species 1 and 2, respectively.
KINETICS WITH ENZYME IN FREE FORM
The overall reaction for FAME production is presented by Fjerbaek, [34]:
Previously, it was indicated that the enzymatic process for FAME, based on production from the Ping Pong Bi Bi mechanism with competitive inhibition of alcohol, uses Equation 14 [6],[19],[35]:
For the development of the kinetic model with enzyme in free form, a system of equations that represent a hydroesterification and alcoholysis process in parallel was used, generated through the Ping Pong Bi Bi mechanism [7],[8].
The mechanism comprises three basic assumptions:
(1) The reaction rate is slow enough for the mass transfer limitations to be negligible.
(2) All fatty acids released can be grouped and treated as a single constituent (F).
(3) The inhibition reaction of the enzymatic active site by alcohol follows a mechanism of competitive inhibition.
The resulting expressions for the reaction rates involved in hydrosterification and alcoholysis process in a parallel mechanism are described by Cheirsilp [7]:
This mechanism has 12 unknown parameters, which are V mT , V mD , V mM , V eT , V eD , V eM , V eEs , K mT , K mD , K mM , K mF and K I .
This system of equations (Equations 4-12) has advantages over Equation 3 because it makes it possible to differentiate each of the intermediates and by-products that are part of a real process of enzymatic FAME production, unlike the simplified model, which only considers the overall reaction. In this model, it was assumed that the initial concentration of water in the medium relates to the soluble concentration. For the simulation of the model, discontinuous methanol feed was taken into account at three different times (0, 3 and 6 hours) until a 4.10:1 methanol: oil molar ratio was achieved.
INTERNAL MASS TRANSFER
After preparing the mathematical model for the process and identification of kinetic parameters without further effects of mass transfer limitations, the next step was to identify the mass transfer limitations for an immobilized enzyme system.
Figure 1 shows the adsorption isotherm of the immobilization support. The elongated form of the hysteresis cycle could correspond to a mesoporous material with very uniform cylindrical pores, but could also relate to mesoporosity generated in the interparticle spaces [36]. The average pore size of the mesoporous, which was calculated using the DFT method, was 4nm. On other hand, the Thermomyces lanuginosus lipase has a volume of 5 nm x4.5 nm x3.5 nm [37]. Thus, it was deemed that the immobilization of the enzyme occurred only on the outside of the pores and in the interparticle space of the material [36]. Therefore, in this case, internal limitations by mass transfer were considered negligible.
EXTERNAL MASS TRANSFER
The rate of FAME production using the immobilized lipase was not governed solely by the reactions of the substrates at the solid-liquid interface. This was evident when the percentage of FAME obtained by using the enzyme in free was compared by using the enzyme in immobilized form, as illustrated in Table 4.
Because the internal mass transfer limitations were negligible, the decrease in the percentage of FAME obtained was attributed only to the limitations caused by external mass transfer to the immobilization support.
According to work by Al-Zuhair et al [2]., the limitations of external mass transfer when using the enzyme in immobilized form are due to the fact that the glycerol that is produced as the reaction proceeds, is deposited on the surface of the support, forming a layer that grows over time [2]. The rate of diffusion of the substrates (triglycerides, methanol and water) is limited through the glycerol stagnant layer surrounding the immobilization support. It is also assumed that these mass transfer limitations do not apply to intermediate species produced in the reactions (diglycerides, monoglycerides and free fatty acids), because once they are produced, they are immediately available at the periphery of the enzyme to react.
Under these conditions, the rate of consumption of triglycerides, methanol and water in the oleic phase can be expressed by the following equations [2]:
Parameter σ makes it possible to modify the mass transfer coefficients as the reaction time increases, representing the growth of the glycerol layer around the immobilization support. The value of parameter σ takes the value of 1 at time zero and the value of zero at infinite time, in accordance with the following equation [2]
The mass transfer coefficients k¡ were calculated in a manner similar to those for enzymes in free form, assuming that the immobilization support has a spherical shape. The system for calculating the mass transfer coefficients of each species i was:
The experimental tests to ascertain particle size distribution of the support were not carried out in this study. It was deemed that the diameter of the immobilization support was 200 nm and spherical in shape, according to the supplementary information presented in the paper by Loganathan et al., used in the synthesis of the immobilization support [38]. Experimentally, it was found that the value for density of the porous support was 572.3 kg/nr [21]. Further, to calculate the Reynolds number, the immobilization support was assumed to be a free-moving sphere inside a stirred vessel. The Archimedes number (Ar) was calculated for this situation and then the Reynolds number (Re) was calculated according to the value taken from the Archimedes number [32],[33], The Schmidt number (Sc) was then calculated, considering the diffusivity of triglycerides, methanol, and water in the glycerol.
Once the equations describing the mass transfer rate from the oleic phase to the solid-liquid interface were obtained, the next step was to couple these expressions to the reaction rate system that was previously illustrated for the enzyme in free form. The reactions were developed over the solid-liquid interface and the concentrations T i , Al i and W i , change according to the following Equations [2]:
In Equations 17, 18 and 19, the first terms enclosed in brackets correspond to mass transfer rates, while the second terms enclosed in brackets correspond to reaction rates. The other chemical reactions that are part of the reaction mechanism were modified only in terms of the concentrations at which the reactions were carried out, I.e., the concentrations at the Interface. Thus, [TG] was changed by [T i ], [Al] by [Al i ] and [W] by [W i ], corresponding to the concentrations at the interface for the triglycerides, alcohol and water respectively.
4. RESULTS
RESULTS FOR THE CALCULATION OF THE LIMITATIONS BY MASS TRANSFER WITH ENZYME IN FREE FORM
According to Equations 11 and 12, the value of methanol diffusivity in the oil was calculated as:
The calculated value of the mass transfer coefficient K L1 was:
Finally, based on Equation 2 the transfer rate of water from the aqueous phase to the oleic phase was calculated as:
Assuming that the mass transfer of water was the limiting step of the process with enzyme in free form, it is possible to analyze what the hypothetical behavior would be for the chemical species that appear in the process that's associated with water consumed by the reaction. In the case of free fatty acids, whenever an acylglyceride hydrolysis reaction occurs, a mole of free fatty acids is generated for every mole of water consumed. Thus, a simulation was performed to observe the hypothetical behavior of the free fatty acid concentration, indicating what would happen to the concentration thereof if the water transfer rate was the limiting step in the process, which is illustrated in Figure 2.
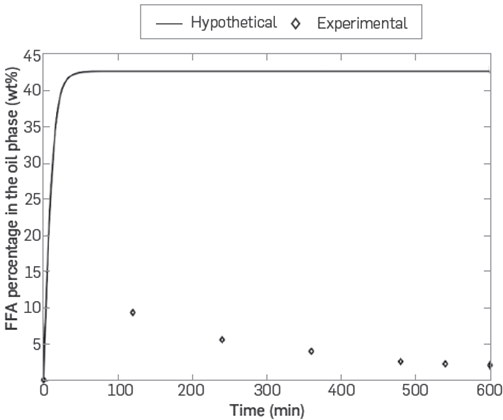
Figure 2 Hypothetical percentage of free fatty acids for a process controlled by the mass transfer rate for water in terms of experimental percentages
In Figure 2, it is seen that the hypothetical curve for the mass percentage of free fatty acids in the oleic phase (line) is above the experimental values reported in the kinetic tests (squares). This means that the reactive process for the production of fatty acids dominates the mass transfer process for water. Therefore, the mass transfer limitations are negligible with respect to the aqueous phase to the oleic phase using the enzyme in free form.
RESULTS FOR THE MODEL DEVELOPMENT WITH ENZYME IN FREE FORM
Table 3 shows the values of the parameters that fit best with the model and the accumulated error. The graphs produced by the simulation and fit of the model are Illustrated in Figure 3.
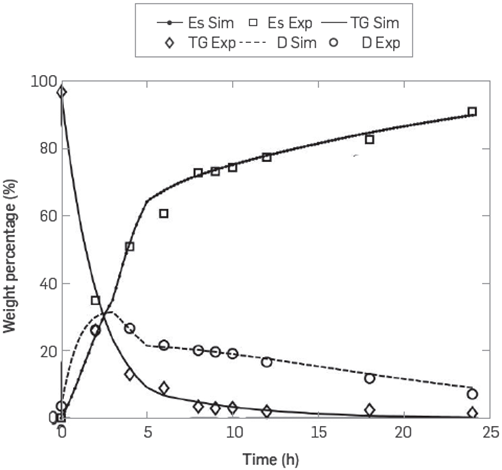
Figure 3 Comparison between the calculated concentrations (lines) and experimental data (symbols) of the species esters (Es), triglycerides (TG), and diglycerides (D) for the enzymatic production of FAME with enzyme in free form
Figure 3, which was produced by the simulation, illustrates that the continuous lines follow the tendency of the experimental data observing greater lags for the curve of methyl esters at 2 and 6 hours, for triglycerides at 2 hours and for diglycerides at 24 hours [7] It is Important to highlight the slope change for the enzyme process in free form at the 5-hour mark. This fact is interpreted as the preference of the enzyme to continue the FAME production process through esterification and not through simultaneous alcoholysis and esterification. The accumulated error obtained for the kinetic model was 4.491. This means a better fit for the hydroesterification and alcoholysis model than the model published by Cheirsilp et al. which was 34.39 [16].
The kinetic parameters obtained, as well as the concentration profiles of the product species of the simulation, serve as valuable information for the calculation of the limitations of external mass transfer imposed by the immobilization support, since they are clearly associated to the reaction processes.
VALIDATION OF THE MODEL WITH ENZYME IN FREE FORM
The model was validated by calculating the relative error rate for the prediction of FAME content during 9 hours, at a temperature of 34 °C, 4.10:1 methanol: oil molar ratio, 0.1454 mg protein/g oil, 2 % weight of water relative to the oil, and 200rpm working conditions.
These experimental conditions were produced in triplicate. Table 4 shows the results of the experimental measurements and the standard deviations of FAME content.
The relative error rate for FAME e(%) was calculated by González [29] as:
The model generated the FAME concentration of 73.47 %, while experimentally it was found that the FAME concentration under these same process conditions resulted in a value of 73.36±0.33%, The relative error rate for FAME was 0.14 %
COMPARISON OF ENZYME PERFORMANCE IN FREE AND IMMOBILIZED FORM
The percentage of FAME obtained when using the enzyme in free form was compared to the enzyme in immobilized form for the FAME production process under the same processing conditions (34°C, 0.1454 mg protein/g oil, 4.10:1 methanol: oil molar ratio and 9 hours' reaction), as shown in Table 4. It was found that the percentage of FAME obtained decreased from 73.36±0.33 % when using the enzyme in free form, and to 47.03±4.43 % when using the immobilized enzyme. After performing a comparison of means with a significance level of 0.05, the decrease in FAME percentage was found to be statistically significant at the 95 % level and was attributed to the external mass transfer limitations imposed by the immobilization support [39].
RESULTS FOR THE ELABORATION OF THE MODEL WITH ENZYME IN FREE FORM
The mass transfer coefficients calculated for triglycerides, methanol and water from the oleic phase to the solid-liquid interface were:
The value of parameter a in Equation 27 was adjusted in accordance with experimental data for the immobilized enzyme system at a value of 2.61 h-1. The expected dynamic behavior for the concentrations of FAME, monoglycerides, diglycerides, triglycerides and free fatty acids in the oleic phase is shown below.
Figure 4 illustrates a fast rate of triglyceride consumption in the first instants of the simulation (0.2 hours), while the increase in the concentration of the other chemical species is slow. This was attributed to the mass transfer phenomenon in the early stages of the process. Subsequently, it was observed that triglyceride consumption began to decrease progressively, while on the other hand it increased in the concentration of the other species, most likely due to the formation of the layer of glycerol and the progress of the reactions.
VALIDATION OF THE MODEL WITH ENZYME IN IMMOBILIZED FORM
As observed in the kinetic model with the enzyme in free form the validation of the model with enzyme in immobilized form was performed by calculating the relative error rate for the prediction of the FAME content during 9 hours and under the same process conditions. In this case, a FAME content of 47.03±4.43 % was obtained, while the FAME content predicted by the model was 47.04 %. Thus, the relative error rate was 0.026 %.
CONCLUSIONS
After analyzing the mass transfer limitations involved in the FAME production enzymatic process separately, it can be concluded that the mass transfer limitations of the aqueous phase to the oleic phase using the enzyme in free form were negligible, as are the limitations by mass transfer to the interior of the support using the enzyme in immobilized form.
Moreover, it was possible to fit a kinetic model to an enzyme in free form process, with a cumulative error of 4.49. This indicates that the cumulative error obtained for the free enzyme model had a better fit than the original model based on literature, which had a value of 34.39. The model was validated by predicting the FAME content obtained experimentally using the enzyme in free form for 9 hours. The prediction error of the kinetic model with enzyme in free form was 0.14 %.
Finally, a model was developed for the prediction of kinetics with the enzyme in immobilized form, in which mass transfer Limitations were completely attributed to the external part of the immobilization support, due to the formation of a layer of glycerol around itself. The model was validated by predicting the FAME content obtained experimentally using the enzyme in immobilized form during the 9 hours. The prediction error for the kinetic model with immobilized enzyme was 0.03 %.