1. INTRODUCTION
Most oil fields have an accelerated rate of production and depletion of reserves due to high demand and a low global supply of hydrocarbons. This situation demands developing and improving conventional EOR techniques with tolerance to adverse high temperature and salinity (HTHS) conditions [1]-[3]. For decades, injection of water-soluble synthetic polymers, generally polyacrylamides [4], is the more frequent flooding technique used worldwide to improve oil efficiency sweeping and oil/water mobility control in the reservoir with oil recovery percentages between 5-30% [5]-[7]. However, synthetic polymers, specifically partially hydrolyzed polyacrylamides (HPAM), have several disadvantages that limit their application in HTHS reservoirs. The use of conventional HPAM is not feasible at temperatures higher than 120°C, brines with organic traces, and total dissolved solids (TDS) greater than 5%w/w, high shear rates ( > 5,000 s-1), and low tolerance to oxide-reduction conditions [8]-[10]. On the other hand, from an environmental point of view, synthetic polymers injection in EOR applications involves significant amounts of raw material (i.e., acrylates) that result undesirable in an eco-friendly scenario. Hence, the industry's efforts are currently focused on the research, development, evaluation, and application of highly tolerant and stable polymers, mechanically, thermally, and chemically, with a green impact at field-scale [11],[12].
The study and application of polysaccharides for EOR applications in HTHS reservoirs are gaining interest in the oil industry. Some of the most studied products include:
Xanthan and diutan gums,
Hydroxyethylcellulose (HEC) and carboxymethylcellulose (CMC),
Schizophyllan and Scleroglucan.
A recent study suggests that these chemicals are considered promising in polymer flooding EOR processes, as replacement of HPAM polymers. The latter is based on the fact that biopolymers show high tolerance to mechanical degradation and high viscosities at lower concentrations as compared with conventional polymers [13].
Xanthan gum has been widely used in EOR processes as it exhibits high resistance to electrolytes and is mechanically stable at high shear stresses at temperatures below 90°C. Low purity, injectivity, and high bacterial degradation are among other limitations [14]. It is worth noting that diutan gum and Schizophyllan can tolerate HTHS conditions (up to 150 °C) due to its higher pseudo-plasticity and mechanical stability [15].
Recently, Schizophyllan, a neutral polysaccharide obtained in liquid form, was first tested at field conditions (T>135 °C, TDS of 186 g/L, and 60 ppm Fe+2) in Germany [16],[17]. This biopolymer showed good mechanical stability at high shear stresses (35,000 s-1). The injectivity of a 350 ppm solution was stable (200-2,000 mD formation) with steady resistance factors (RF) throughout the test. Schizophyllan also showed better rheological performance compared to xanthan gum and polyacrylamides. Additionally, as a lesson learned, they required nitrogen blanketing due to the transport and preparation of the biopolymer before injection (the oxygen-free environment must be 100% secured). Finally, the injection of this biopolymer showed a 25% increase in oil recovery as compared to water injection. The authors reported that the proximity between the injector and the producing wells is a good indication of the effect of the biopolymer for the assessed period (6-7 months), followed by rigorous monitoring and sampling throughout execution of the pilot [17]. However, the standard selection criteria of biopolymers (i.e., solubility, injectivity, adsorption, etc.) is not well documented in the literature.
The application of Scleroglucan as an active agent in EOR processes emerges as an environmentally friendly alternative for harsh conditions in reservoirs with high salinity, temperature, and shear stresses, due to its rigid, triple helix structure [12],[18]. Several authors have reported the excellent performance of Scleroglucan in adverse reservoir conditions [19],[20], stability, and high retention for three months at 80°C in high salinity [21]-[23]. Scleroglucan shows great potential with better performance as compared to xanthan gum and polyacrylamides in terms of thermal, chemical and mechanical stability, and oil recovery [12]. Recently, Scleroglucan was evaluated at a laboratory scale for the Adena field reservoir conditions, Colorado, United States. Stability tests were run for temperatures up to 115°C and 180 days, shear rates of 175,000 s-1 and Fe2+ and H2S content of 500 ppm and 200 ppm, respectively. Adena field reservoir temperature is 82°C, and the average rock properties are 19.7% porosity (Ø) and permeability (K) of 805 mD. Main fluid properties are crude oil gravity of 42°API and brine of 8,000 TDS (Total Dissolved Solids), and 35 ppm TSS (Total Suspended Solids) [19],[20]. The concentration determined for these conditions was 300 ppm of the biopolymer to achieve the target viscosity of 1.4 cP. The static evaluation results reflect that the Scleroglucan showed filtering capacity similar to that of water, and stability after 90 days at 82°C without adverse effects on viscosity. Based on the foregoing, this study compares the performance of Scleroglucan (SG) and a commercial sulfonated polyacrylamide (ATBS), based on a laboratory evaluation at static conditions in controlled systems. The purpose of this study is to identify the best polymer candidate for a specific case of a Colombian heavy oilfield (100°C and 3,800 ppm TDS) in terms of stability by thermal (50°C and 100°C up to 180 days), chemical (different ionic conditions) and mechanical (up to 275,000 s-1) effects.
2. EXPERIMENTAL DEVELOPMENT
MATERIALS
A commercial Scleroglucan (SG) of molecular weight (MW) ≈4-5MMDa and a sulfonated polyacrylamide (ATBS-25%) with MW ≈13MMDa, were used in this experimental study. For the preparation of the synthetic brine, 2.9674 g/L NaCl, 0.13 g/L KCl, 0.239 g/L MgCl2·6H2O, and 0.583 g/L CaCl2·2H2O in type II water (pH≈6) were used.
METHODS
THERMOGRAVIMETRIC ANALYSIS (TGA)
The mass conversion of polymers in an inert atmosphere was determined using a Q50 thermogravimetric analyzer (TA Instruments, Inc., New Castle, DE) under N2 flow at 100 mL/min with an optimizing heating rate where sequence programming is started at 30°C with 5°C/min up to 800°C. The procedure consists in weighing a specific amount in the sample holder due to diffusion effects. The Q Series- Q500-TGA commercial software is used to export the analysis data.
PREPARATION OF POLYMER SOLUTIONS
Synthetic polymer solutions at a fixed concentration of 2,500 mg/L were prepared according to API-RP-63 [24]. SG solutions were prepared following the practices recommended by the supplier [20], considering agitation, total solubility time, and hydration to ensure homogeneity, stability, and avoid possible negative effects [25]. First, stir the synthetic brine with a propeller-type agitator at speed enough to generate a vortex of 1/3 of total volume, then sprinkle the biopolymer in the vortex shoulder continuously. Second, stir the mixture at 800 rpm for 10 minutes and heat the solution until it reaches 40 °C. Third, stir the biopolymer solution in an immersion blender (ultra-turrax) at 20,000 rpm for 5 minutes. Lastly, observation analysis, turbidity, and transmittance measurements are required for quality control of a biopolymer solution.
RHEOLOGICAL TESTS
Rheological measurements for polymer solutions were performed using a rheometer Anton Paar MCR 702 (Anton Paar GmbH, AUT) with the concentric cylinder's geometry, equipped with a Peltier temperature device and pressure cell in a nitrogen atmosphere. Each measurement was performed in a range of shear rates of 4-400 s-1, temperatures of 80-100°C, and pressure of 530 psi. For analysis and treatment of rheometer data, a RheoCompass software was used. A DV2T Viscosimeter (Brookfield Ametek, USA) was used for static measurements at a constant shear rate (7.3 s-1) using UL and DIN adapter spindles following standard practices [24].
HYDRODYNAMIC MOLECULE SIZE
The hydrodynamic molecule size was obtained by dynamic light scattering (DLS) measurements using a Zetasizer ZS (Malvern Panalytical, UK) after agitation for 15 min at 30°C and 60°C. Polymer solutions were prepared in synthetic brine of NaCl (0, 0.01, 0.1, 1, and 10%w/w) to determine the salt concentration effect. Parameters such as viscosity, refractive index (1.3335-1.3366), absorbance (0.02-0.06), and wavelength (195 and 285 nm) were measured for each sample.
CHEMICAL STABILITY: ELECTROLYTES EFFECT
The cationic effects of Na+, Ca2+, and Mg2+ were evaluated for both polymers in aqueous chloride salts. Viscosity measurements were carried out in a viscosimeter at 7.3 s-1 and temperatures of 30, 50, 80 y 100°C. Viscosities at temperatures above 60°C were tested in the rheometer.
THERMOCHEMICAL STABILITY
The polymer solutions were assessed at different temperatures (30°C to 100°C), as described in the rheology test. Also, polymer solutions in the presence of Fe2+ (0 and 3 ppm) were exposed to high temperature (105°C) for six months. Each sample is prepared and embased in airtight bottles in a vacuum chamber, and then, the bottles are exposed at 105°C for 180 days in a heating oven. Last, viscosity measurements for each container are monitored every 30 days at 7.3 s-1 in an inert atmosphere (0 ppm O2).
FILTERABILITY TEST
Tests of Filterability ("filter") ratio (FR) and cumulative filterability of polymer solutions were carried out according to the API-RP-63 method [24]. For the FR, nitrocellulose membrane filters of 1.2 μm and 5 μm (Merck KGaA, Germany) were used. The accumulated filtration time was recorded for every 100 mL of filtrate until 300 mL, at a constant pressure of 30 psi. For the cumulative filterability test, a 5 μm filter was used, recording the accumulated filtration pressure for 500 mL at a continuous rate of 2 mL/min and 30°C.
MECHANICAL STABILITY
Mechanical degradation tests of the polymer solutions were carried out following the technical test instructions of API RP63 [24] recommended practices. This procedure is performed to simulate the flow conditions of the polymer solutions through the surface facilities and the mechanical well state. In this test, the shear rate evaluated ranged between 5,000 and 275,000 s-1. Mechanical degradation is carried out flowing the pressurized polymer solution through a capillary tube at different flow rates obtained for each pressure recording time and weight of the sheared fluid. Each viscosity of the sheared solution is measured at 7.3 s-1 and room temperature. The shear rate (γ̇) of the polymer solution is calculated based on the flow rates (Q) and the internal radius of the capillary (R) according to Equation 1:
MODELING
RHEOLOGICAL BEHAVIOR
The Carreau-Yasuda model [26] includes rheological parameters for non-newtonian fluids such as relaxation time ((λ), related to the time for the polymer system to return to equilibrium in response to a disturbance. Intrinsic parameters, n and α are related to the transition behavior, and the viscosity parameters (η 0 and η ∞ ) indicate the fluid behavior at zero and infinite shear rate. The Carreau-Yasuda model is expressed as follows [26],[27]:
Where η (cP) and γ̇ (s-1) are the viscosity and shear rates, respectively. The root-mean-square error (RSME%) was used to estimate the proper fit of the model used.
3. RESULTS
THERMAL CHARACTERIZATION
The weight loss (%) and its conversion as a function of temperature for both solid polymers at inert atmosphere are shown in Figure 1. The behavior of both materials corresponds to a mass loss proportional to the increase in system temperature. Figure 1a shows the thermogram for the Scleroglucan. The most significant mass reduction of the material was observed in temperature ranges of <100 °C, 250-350 °C and 450-500 °C, respectively. Each of these weight losses corresponds to the loss of surface-adsorbed water, C02 from carboxyl groups (COO-) decomposition [28] depolymerization reaction [29], and the volatile products of lower molecular weight [30],Figure 1b presents the TGA results for the sulfonated polyacrylamide. In this case, four regions with high mass conversion were registered in temperature ranges of <100 °C, 350-380 °C, 400-450 °C, and 550-650 °C, respectively. These mass reductions are attributed to the breaking of the copolymer bonds that gives rise to the release of water (≤100 °C), loss of structural water by dehydroxylation (≤200 °C), and loss of NH3, S02, CO, and CO2 groups [31,32], In both cases, the mass loss at 600°C indicates significant percentages of total degradation (up to 100%). However, the higher thermal stability of the sulfonated polyacrylamide can be attributed to structural and chemical differences (i.e. presence of functional groups -COO-, -CH2-SO3- CONH2, etc.) compared to SG [31]. Further, the percentage of moisture for the scleroglucan and the sulfonated polyacrylamide at 100 °C in an inert atmosphere correspond to 11% and 9%, respectively [12].
RHEOLOGICAL BEHAVIOR OF POLYMERIC SOLUTIONS
Figure 2, presents the viscosity behavior of SG and sulfonated polyacrylamide as a function of the shear rate. These tests were run for different polymer concentrations prepared in synthetic brine (TDS 3,800 ppm) at 100 °C in a pressurized nitrogen system. The viscous behavior of both polymers tends to increase proportionally with the concentration. Nonetheless, SG exhibits similar viscosities at lower concentrations as compared to the sulfonated polymer due to its configuration and structure. Both polymer solutions show a non-Newtonian behavior. The rheological behavior of both polymer solutions has a shear thinning type due to viscosity reduction with the shear rate increase. However, SG exhibits higher viscosity than the sulfonated polyacrylamide due to its rod-like structure compared to the branched configuration of the ATBS polymer Additionally, the thermal resistance of the biopolymer generates higher stability by the retention of water molecules in their dense structure [12]. These properties represent an optimum scenario for polymer injection. Specifically, the SG polymer solution shows better viscosity performance reducing the concentration up to 3 times to obtain the same target injection viscosity (17 cP) vis-à-vis sulfonated polyacrylamide.
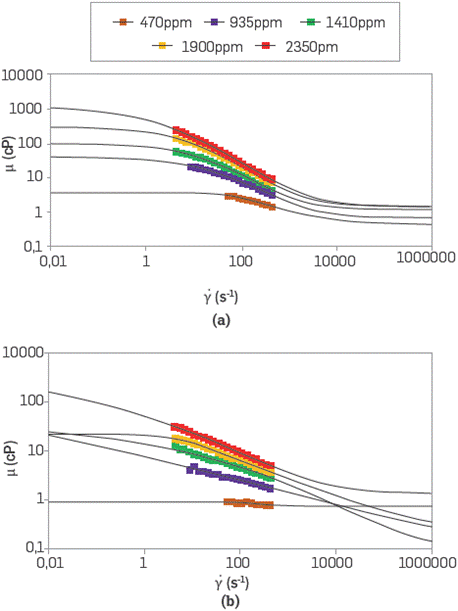
Figure 2 Rheological behavior of (a) scleroglucan, and (b) sulfonated polyacrylamide solutions for different polymer concentrations prepared in synthetic brine (TDS 3,800 ppm) at 100 °C.
The Carreau-Yasuda model parameters for the rheological behavior of polymer solutions are shown in Table 1. SG viscosity parameters at zero and infinity times (η 0 and η ∞) are higher than the sulfonated polyacrylamide solutions. The relaxation times (λ) are higher for Scleroglucan due to its low viscoelasticity compared to polyacrylamide [33]. For both polymer solutions, there are three regions with pseudo-plasticity indices (n) less than one (1), common from a shear-thinning fluid [34], where a first region or first plateau corresponding to viscosity at low shear rates are higher for all SG concentrations. The second region or pseudo-plasticity zone is similar for both polymers. Nonetheless, the ATBS is slightly better due to the higher viscoelasticity behavior of branched polymer chains in comparison with the rod-like structure of scleroglucan [35].
Table 1 Carreau-Yasuda model parameters for the rheological behavior of scleroglucan (SG) and sulfonated polyacrylamide (ATBS) solutions at different polymer concentrations in synthetic brine (3,800 ppm TDS) at 100 °C.
Parameter | SG | ATBS | ||||||||
Concentration (ppm) | ||||||||||
470 | 935 | 1,410 | 1,900 | 2,350 | 500 | 1,000 | 1,500 | 2,000 | 2,500 | |
η0 | 3.5302 | 41.730 | 97.067 | 305.920 | 1,253.20 | 0.886 | 1.777 | 21.822 | 39.158 | 542.090 |
η∞ | 0.4187 | 0.6869 | 1.1918 | 1.4287 | 1.5296 | 0.017 | 0.100 | 0.110 | 0,186 | 1.312 |
λ | 0.0152 | 0.0245 | 0.0648 | 0.1758 | 0.3739 | 0.001 | 0.010 | 0.011 | 0.099 | 0.228 |
α | 1.6744 | 0.5389 | 0.6653 | 0.7149 | 0.4838 | 0.111 | 0.139 | 0.182 | 1.269 | 1.161 |
n | 0.4161 | 0.0001 | 0.0342 | 0.0840 | 0.0228 | 0.051 | 0.143 | 0.443 | 0.601 | 0.961 |
The last region (second plateau), corresponding to high shear conditions, shows higher viscosities for SG polymer due to low mechanical and thermal degradation effects. However, the final viscosity in both cases is similar to water viscosity. However, scleroglucan viscosities are higher than 1 cP at the infinity shear rate due to neutral nature and rod-like structure after the shear effect.
In this case, 17 cP at 100 °C it was determined as target viscosity considering the oil/water mobility ratio close to 1. For SG, the target viscosity of 17 cP reached with the 2,500 ppm of the ATBS polymer is achieved with 935 ppm at the same temperature and salinity conditions. Figure 3 shows the polymer viscosities as a function of polymer concentration in synthetic brine (TDS 3,800 ppm) at 100 °C. It should be noticed that the SG viscosification potential in high-temperature conditions is significant at the polymer concentration increases. Therefore, the polymer concentration of SG and sulfonated polyacrylamide compared in the different static evaluations is 935 ppm and 2,500 ppm, respectively.
THERMOCHEMICAL STABILITY
Scleroglucan and sulfonated polyacrylamide solutions were assessed at different temperatures (30 °C to 100 °C), as shown in Figure 4. The viscosity behavior of SG outperforms the sulfonated polyacrylamide system within the range polymer concentration (470 to 2,500 ppm), and temperatures (30° to 100 °C) are evaluated. The ATBS polymer studied shows a lower viscous range (25.8 cP at 100 °C) with a higher polymer concentration (2,500 ppm).
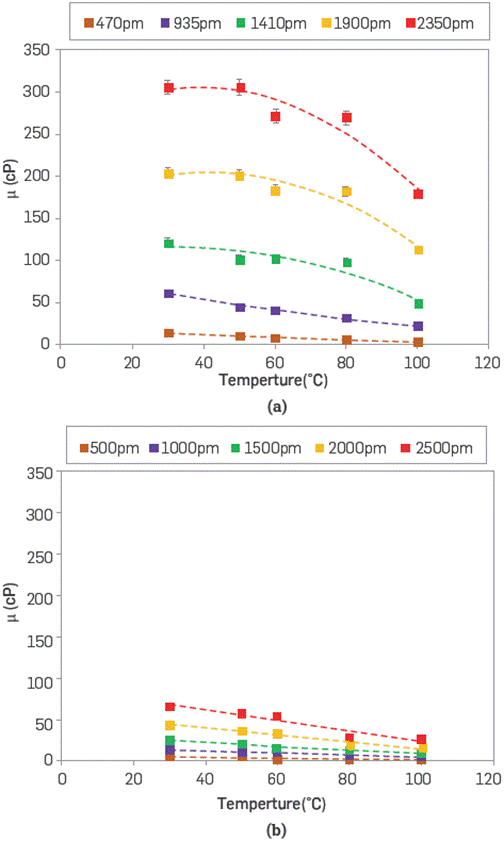
Figure 4 Viscosity behavior of (a) SG and (b) ATBS polymers at different concentrations (470-2,500 ppm) in synthetic brine (TDS 3,800 ppm) and temperatures (30° to 100 °C) at 7.3 s-1.
The thermochemical stability of polymer solution represents a critical criterion for polymer selection in polymer flooding. Harsh reservoir conditions can negatively impact polymer viscosity due to intermolecular forces and polymer chain interactions, decreasing it due to electrolytes and high-temperature effects [35],[36], The SG and ATBS polymer solutions were also evaluated over time (0 to 180 days) at 105 °C with polymer concentration (935 ppm and 2,500 ppm) to reach the target viscosity of 17 cP in the presence of Fe2+ in an inert atmosphere. SG showed a steady viscosity behavior during 180 days with losses below 1 % from the initial viscosity. The ATBS polymer lost less than 20 % of its initial viscosity after 90 days, with only the temperature effect under anoxic conditions, as reported in the literature [37], The behavior of SG solutions (935 ppm) and sulfonated polyacrylamide (2,500 ppm) under different pH range at different temperatures are shown in Figure 5. The chemical nature of each system plays a fundamental role in their viscosity behavior, depending on the solution pH. In the case of scleroglucan, which has a neutral character, the viscosity is not adversely affected when the system is hugely acidic (pH~2) or moderately basic (pH~10) However, there is evidence when the pH is higher than 13, the viscosity is drastically reduced due to the denaturation of the triple helix structure with high alkali concentrations [38],[39], The ATBS polymer, which is a polyelectrolyte, is affected by the pH variation which makes it dependent on the electrical charges, and its stability decreases when it is an acidic system.
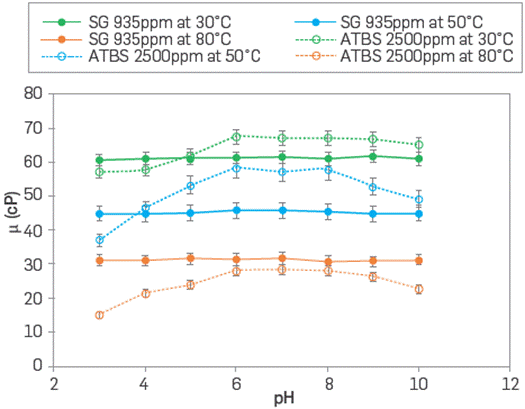
Figure 5 Viscosity behavior of SG (935 ppm) and ATBS polymer (2,500 ppm) as a function of pH at different temperatures (30° to 80°C) in synthetic brine (TDS 3,800 ppm) and 7.3 s-1.
The polymer solution system was also assessed under different ionic strength (0.043, 0.086, 0.171, and 0.342) with the concentration of monovalent and divalent ions (Na+, Ca2+, and Mg2+) present in the formation water to observe the solubility, hydration, and viscosity depending on the reservoir temperature. The viscosity of both polymer solutions as a function of NaCl concentrations (0 to 5 %w/w NaCl) is shown in Figure 6. A similar trend is evident in both polymers after 0.15 %w/w. Scleroglucan exhibits higher stability, from 0 to 5 %w/w of NaCl, which confirms its high tolerance to high salinity and temperature, as reported in the literature [25],[40].
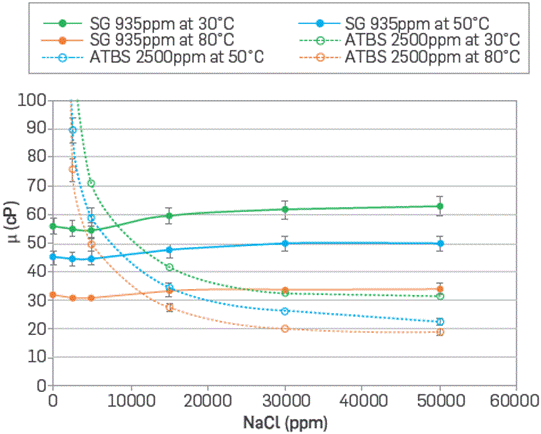
Figure 6 Viscosity behavior of SG (935 ppm) and sulfonated polyacrylamide (2,500 ppm) at different temperatures (30° to 80 °C) and NaCl concentrations (0 to 50,000 ppm) at 7.3 s-1.
Figures 7 and 8 show the effects of divalent cations (Ca2+ and Mg2+) on SG and ATBS polymer viscosities. Figure 7, shows the viscosity behavior of both polymer systems in the presence of different concentrations of CaCl2. The scleroglucan viscosity is stable with the calcium concentration increasing in the solution for all temperatures evaluated. Conversely, the sulfonated polyacrylamide solution showed an apparent decrease in viscosity, increasing calcium concentration and temperatures. This case confirms the higher sensitivity of polyacrylamide by divalent ions due to the reduction of polymer chain entanglement [41].
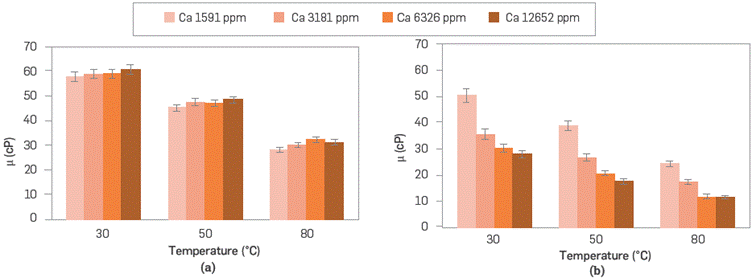
Figure 7 Viscosity behavior of (a) scleroglucan (935 ppm) and (b) sulfonated polyacrylamide (2,500 ppm) in synthetic brine with different ionic strength of Ca2+ at temperatures of 30°, 50° and 80°C and 7.31 s-1.
The behavior of the polymer solutions in the presence of Mg2+ ion shows a viscosity reduction effect in both polymers. Although both polymer viscosities decrease with the increase of Mg2+ concentration, SG seems to be more tolerant than the ATBS polymer at the conditions assessed (Figure 8). The impact on the viscosity reduction is the result of the divalent ion interaction with the amine groups present in the branched-chain of the sulfonated polymer [31].
HYDRODYNAMIC SIZE BEHAVIOR
Scleroglucan and sulfonated polyacrylamide configurations in the aqueous phase are detected as spherical shape by the DLS technique, as shown in Figure 9. In this case, it is considered that SG and ATBS polymer molecules can be circular or linear organization [42],[43], depending on the interaction between molecules when increasing the concentration, temperature, salinity, pH, and other. This structural behavior determines the polymer viscosity in solution; however, the accuracy of the DLS technique for this type of macromolecules is limited by laser angles, absorbance, refractive index, dielectric constant, and viscosity [43], In this study, DLS measurements were used to relate the behavior of polymers hydrodynamic size and its viscosity with the variation of NaCl concentrations.
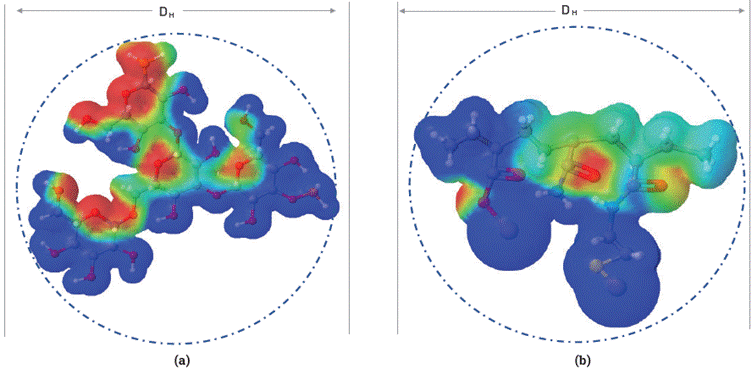
Figure 9 Hypothetical electrostatic potential and hydrodynamic shape of a structural unit of a) scleroglucan (SG) and b) sulfonated polyacrylamide (ATBS) in aqueous phase. The red color indicates regions of high electron density, blue regions represents low electron density, and yellow and green represent a homogeneous electron balance.
The hydrodynamic behavior of polymer solutions shows high stability when salinity is less than 1 % of NaCl. Nonetheless, after the aggregates, the entanglement reduces the average size of sulfonated polyacrylamide, where this effect is directly proportional to the viscosity system, reducing it more than 50 % in both temperatures evaluated. Both polymers have similar viscosity and different aggregates size within 1 % and 10 %wt of NaCl. The sulfonated polymer coils up at high NaCl concentration reducing the hydrodynamic size and interaction of the polymer chains, causing a reduction in the viscosity [44].
Regarding SG, the viscosity and hydrodynamic behavior are less significant with the electrolyte charge and temperature effects, where viscosity reduction is less than 20 % (Figure 10). Both polymers are governed mainly by factors such as electrostatic interactions [45], Van der Waals forces [46]0[47], hydrogen bonding [48],[49], hydrophobic interactions [50], and excluded volume effects [51] that govern the hydrodynamic behavior of the aqueous configuration. Hydrogen bonds could be the primary interaction in the stabilizing intra-molecular shape or formation of junction zones for scleroglucan due to helices and β-sheets in its structure [52].
As regards ATBS, the electrostatic interactions and hydrogen bonds being are the main dominant effects due to the anionic nature and the intermolecular entanglement [36], This hydrodynamic size (DH) behavior of a polymer solution is a crucial parameter for the understanding of flow resistance or size distribution limits of polymer in terms of flow in the porous medium [5].
MECHANICAL STABILITY
Figure 11a shows the flow time of polymer solutions through a pore-size nitrocellulose membrane of 1.2 and 5 μm, which restrict the flow when the system is pressurized. Both polymer solutions have a filterability ratio between 1 and 1.2, regardless of the time in which the same volume is filtered [24]. SG solutions flow through the filter in less than 1 minute regardless of the membrane pore size and despite the rigid rod-like structure of this biopolymer. On the contrary, the ATBS polymer solutions required 100 minutes to filter the same volume. The structure of this sulfonated polymer corresponds to a set of aggregates that tend to pass through the pore with greater difficulty. This behavior is related to Figure 11b, where the same volume is filtered at a constant flow rate (2 mL/min) in a pore size of 5 μm. In this system, the solution with Scleroglucan has a lower energy expenditure in terms of pressure to be injected compared to sulfonated polyacrylamide in a significant proportion. The aggregate sizes of the sulfonated polyacrylamide (DH <5 μm) tend to trap the space by mechanical effects to the point where the system continually flows at a specific pressure (120 psi). Conversely, SG exhibits a steadier behavior showing a maximum flow pressure of 14 psi after the 80 pore volume is injected (PVI). However, it is still much lower than that required by the synthetic polymer. Its behavior is perhaps attributable to the rearrangement of the dense rod-structure passing through a linear space.
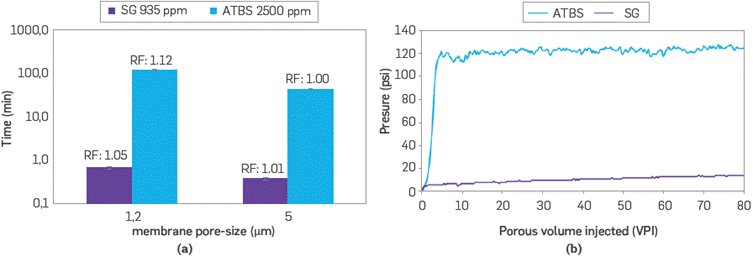
Figure 11 (a) Filterability ratio in nitrocellulose membranes of 1.2 μm and 5 μm pore-sizes, and b) Cumulative filterability ratio in 5 μm filter at a constant flow rate (2 mL/min) of SG (935 ppm) and ATBS (2,500 ppm) polymer solutions in synthetic brine (TDS 3,800 ppm).
For the mechanical degradation of the polymer solutions, each system was evaluated at different shear rates to determine the viscosity losses associated with mechanical degradation. Figure 12 shows the viscosity loss of Scleroglucan and sulfonated polyacrylamide as a function of the shear rate. In this scenario, the viscosity losses of the SG are less than 30 % at shear rates of up to 275,000 s-1. These results confirm the low mechanical degradation results reported in the literature with much lower shear rates (up to 1,000 s-1) [20], However, viscosity losses of the sulfonated polyacrylamide reach up to 80 % of its initial viscosity under the same conditions, which lead to a technical-operational disadvantage. The injection of SG would allow the current operation of conventional waterflooding, the use of flow regulating valves, selective strings, and well spacing without any significant adverse effect on the polymer viscosity as presented in the mechanical stability test when subjected to high cutting rates. Conversely, this advantage over synthetic polymer implies lower resources and equipment in the application of field technology.
CONCLUSIONS
This study compares the performance of Scleroglucan (SG) biopolymer and a commercial sulfonated (ATBS) polymer under different conditions. Overall, SG stands out for generating higher viscosities at lower polymer concentrations and exhibits excellent stability at HTHS and high shear rate conditions than the ATBS polymer evaluated.
The shear-thinning flow behavior of Scleroglucan suggests that it should have good injectivity based on the filter ratio results, even with the rod-type structure. The properties exhibited of this biopolymer make it possible to broaden the range of application restricted oil reservoirs with extreme temperature, salinity, and hardness conditions.
Scleroglucan shows promising properties for EOR applications, including heavy oil reservoirs at high temperature, preserving the viscosity and stability at high salinities (0-5 %w/w), ionic strengths (Na+, Ca+2, and Mg+2), up to 275,000 s-1, temperatures (>80 °C), and wide pH ranges (3-10), tested in this study.