INTRODUCTION
Cyanide is highly toxic to most living organisms. The toxic dose in humans is 1 mg/kg body weight, with higher doses potentially causing death within minutes (Vogel et al, 1981). Despite its toxicity, cyanide is widely used in several industrial processes, including the chemical synthesis of polymers, electroplating, and gold mining (Cummings, 2004); with the latter industrial activity posing the largest environmental hazard (Johnson, 2015). Gold mining practices generate large volumes of cyanide-laden waste effluents, usually kept in dams, which need to be properly contained and remediated. Unfortunately, there are numerous examples of the catastrophic effect of accidental spills from mine waste dams (Henderson, 1995; Rico et al., 2008).
In recent years, global gold price has increased 360 % (Swenson et al., 2011). As a result, gold mining has dramatically increased in developing countries. Gold mining in Latin America has grown rapidly, and by 2010 the region contributed 19% of the total world production (Swenson et al., 2011). Unfortunately, poor control and management of the chemicals used in the process have resulted in spills of cyanide, mercury, and other heavy metals into rivers and soil, causing severe injury to exposed human inhabitants, wildlife, and overall biodiversity (Tarras-Wahlberg et al., 2001). A rapid, cost-effective, environmentally-friendly solution for the treatment of the cyanide wastewater generated by gold mining is urgently needed.
Conventional chemical methods used to treat cyanide wastewater have drawbacks, such as high costs, the need for special infrastructure, and the use of chemical reagents that are themselves environmental hazards (Akcil, 2003). Considering these limitations, biological methods for cyanide degradation represent attractive alternatives (Dash et al., 2009; Gupta et al., 2010). Specifically, the enzymatic degradation of industrial cyanide wastewater by cyanide dihydratase (CynD) a member of the nitrilase superfamily, has been reported as a plausible alternative (Martínková et al., 2015; Park et al., 2017; Thuku et al., 2009). The appeal of using CynD enzymes derives from their ability to rapidly convert cyanide into ammonia and formate without any added cofactors.
Jandhyala et al. (2003), expressed CynD from Bacillus pumilus in recombinant E. coli and purified the enzyme using a histidine tag. The recombinant enzyme displayed maximum activity between 37 °C and 42 °C, was inactive at a pH >8.5, and was greatly inhibited by Hg2+ (Jandhyala et al., 2005). More recently, protein-engineering efforts resulted in CynD mutants and chimeras with increased activity at an alkaline pH and increased thermal stability (Crum et al., 2015; Crum et al., 2016). More recently, a CynD from Flavobacterium indicum was immobilized on agar to develop a potentiometric biosensor for detection of cyanide (Kumar et al., 2018). In spite of the success in improving the catalytic properties of purified CynD enzymes, an even more robust catalyst is required for the bioremediation of gold mining cyanide wastewater.
The use of whole cells engineered to overexpress enzymes of interest for industrial processes is appealing because cellular membranes and the cytoplasm can provide some level of protection to intracellular enzymes against detrimental conditions, such as extreme pH, temperature, and inhibitors; thereby making whole cells more suitable for industrial processes (De Carvalho, 2016; Lin & Tao, 2017; Wachtmeister & Rother, 2016).
Based on this assumption, and findings by Vargas-Serna and Panay (2019), we hypothesized that whole E. coli cells overexpressing CynD could be better suited for degrading cyanide under the harsh conditions present in the cyanide wastewater generated by gold mining.
MATERIALS AND METHODS
Bacterial strains and plasmids
Escherichia coli DH5a was used for plasmid DNA replication and Escherichia coli BL21(DE3) was utilized for protein expression. The Bacillus pumilus C1 cyanide di-hydratase gene sequence (GenBank AF492815) (Jandhyala et al., 2003) with an additional C-terminal hexahistidyl tag was codon optimized for E. coli protein expression (GenBank MH917689), synthesized and cloned in a vector PD451 by DNA 2.0 (Atum, Newark, CA, USA). This plasmid, named PD451-BpumCynD, is available upon request.
Whole cells expressing CynD (E. coli-CynD)
Single colonies of E. coli BL21(DE3) transformed with the plasmid PD451-BpumCynD were grown in LB media for 16 h at 37 °C and 300 rpm. Fresh cultures were initiated by adding a 100-fold dilution of the overnight culture to fresh LB media supplemented with kanamycin. When the OD600nm reached 0.5, the cultures were induced with 0.4 mM IPTG. CynD expression was monitored by SDS-PAGE. Cells were harvested 4 h after induction by centrifugation for 30 min at 20 °C and 5800 rpm using an Eppendorf centrifuge 5804R. Cell pellets were immediately used or stored at -80 °C. Untrans-formed E. coli BL21(DE3) was grown following a similar procedure but without the addition of antibiotics or IPTG. This culture served as a negative control for cyanide degradation.
Cyanide determination
Cyanide concentrations were measured by the picric acid method (Fisher & Brown, 1952) mixing 500 µL of cyanide-containing solution and 500 µL of 0.5 M sodium carbonate and 1% picric acid. This solution was heated at 100 °C for 5 min and the reaction stopped with 3.5 mL of water. Cyanide concentration was determined measuring absorbance at 520 nm utilizing a standard curve ranging between 0 to 4.0 mM cyanide. For samples with concentration > 4.0 mM the assay supernatant was diluted so the absorbance fell within the linear range of the standard curve.
E. coli-CynD activity measurements
For whole cell kinetics, reactions were carried out in a volume of 1 mL, containing 0.1 mg of cells in 20 mM tris (pH 8.0), and varying amounts of cyanide. Initial velocity rates were calculated from the linear portion of plots of cyanide concentration vs time. Kinetic parameters were determined from plots of initial velocities against cyanide concentration fitted to the Michaelis Menten equation. For total cyanide degradation, pellets of E. coli-CynD were brought to an OD600nm of 1.1 in: LB broth for growing cells, 20 mM buffer tris (pH 8.0) for resting cells, and 20 mM tris (pH 8.0) with 5% toluene and 1 mM EDTA for permeabilized cells. Total cyanide degradation was determined after 10 minutes of reaction.
Negative controls included untransformed E. coli BL21 (DE3) and a solution without the addition of cells which was used to account for cyanide loss through evaporation. All of the results represent the average of three independent measurements. Percent cyanide removed was calculated using Equation 1, where [Cyanide0]: is the initial cyanide and [Cyanidef] is the final cyanide concentration.
Activity at basic pH
For total cyanide degradation at alkaline pH, E. coli-CynD cells were resuspended in 20 mM buffer tris (pH 8.0) and the suspension was adjusted to an OD600nm of 1.1. Reactions were carried out in either 20 mM tris pH 8.0, 50 mM glycine pH 9.0 or pH 10.0 in a total volume of 1 mL with 5 mM cyanide and 100 µL of the cell suspension. The activity is reported relative to that at pH 8.0. Total cyanide degradation at each pH was determined at 10 min intervals up to 1 hour.
Optimum Temperature and thermostability
The activity of 0.1 mg of E. coli-CynD was determined in a range of temperatures from 35 °C to 60 °C with five-degree intervals. Assay solutions contained 5 mM cyanide in a total volume of 1 mL, 20 mM Tris (pH 8.0). Activity is reported relative to that at 35 °C. All assays were performed in triplicate.
For thermal stability, E. coli-CynD was adjusted to an OD600nm of 1.1 in 20 mM buffer tris (pH 8.0). The cells were incubated at 42 °C for 75 h. Every five hours the enzymatic activity was determined using 100 µL of the cells. All assays were performed in triplicate.
Heavy metal inhibition
Cyanide removal was assayed in the presence of FeCl3, HgCl2, and NiSO4. E. coli-CynD was adjusted to an OD600nm of 1.1 in 20 mM buffer tris (pH 8.0) and 100 µL were incubated with 200 ppm of the metal for 1 hour. The activity of the metal incubated E. coli-CynD cells was determined in 1 mL assays with 5 mM cyanide and 20 mM Tris (pH 8.0) buffer. Activity is reported relative to E. coli-CynD cells that were not incubated with any heavy metal salts. All assays were performed in triplicate.
Bioremediation of industrial cyanide wastewater
Samples of industrial cyanide wastewater were obtained from a gold processing plant belonging to La Sociedad Minera del Sur S.A., Buenos Aires Cauca, Colombia. Total metal content in the samples was analyzed by inductively coupled plasma mass spectrometry (ICP-MS) by the laboratory SGS, Bogotá, Colombia. The concentration of free cyanide in the industrial samples was determined using the picric acid method.
Whole E. coli-CynD cells were adjusted to an OD600nm of 1.1 in 20 mM Tris (pH 8.0) buffer. From this solution, 100 µl of cells were added directly to 900 µl of the cyanide-containing wastewater and the cyanide content determined after 30 min. Additional reactions were carried out using cyanide wastewater diluted 30-fold with water and either 100 µl or 300 µl of E. coli-CynD. Also, 300 µl of a cell resuspension composed of 200 µl E. coli BL21(DE3) and 100 µl of E. coli-CynD were used as a control for the possible effect of E. coli cellular components on inhibiting constituents present in the cyanide waste solution.
Cell viability in industrial cyanide wastewaters
Permeabilized and non-permeabilized E. coli-CynD cells were added in a 1:10 ratio to 1 mL cyanide wastewater solutions and allowed to grow for 3 days at 25 °C with constant agitation. Ten µL from the solution were plated on LB-Kanamycin agar plates and the colony forming units (CFUs) determined after 2 days of growth at 37 °C. As a control we did a similar procedure but with E. coli-CynD cells grown in LB broth. All experiments were performed in triplicate.
RESULTS AND DISCUSSION
Overexpression of intracellular CynD and kinetics
Recombinant CynD expressed abundantly in E. coli, with maximal expression at 4 hours after IPTG induction. The prominent band at 38 kDa observed in SDS-PAGE gels of total protein content (Figure 1) corresponds to the protein product predicted by the gene sequence (Jandhyala et al., 2003). E. coli-CynD showed saturation kinetics (Figure 2) with values for Vmax of 8.5 µmol min-1 mg-1 and KM for cyanide of 2.1 mM. These values can be compared with those reported for the purified enzyme (Vmax 88 µmol min-1 mg-1 and KM 2.56 mM) (Jandhyala et al., 2005;). The similar KM value for intracellular and purified enzyme suggests that cyanide can rapidly diffuse through the cell membrane, rapidly equalizing inner and outer concentrations, a characteristic that can contribute to cyanide cytotoxicity. With regards to Vmax, comparisons with the purified enzyme are impossible due to the unknown concentration of CynD inside E. coli. Altogether, these values can be compared with other intracellular CynD enzymes like that of F. indicum (Vmax 0.33 µmol min-1 mg-1 and KM 5.25 mM) (Kumar et al., 2018).
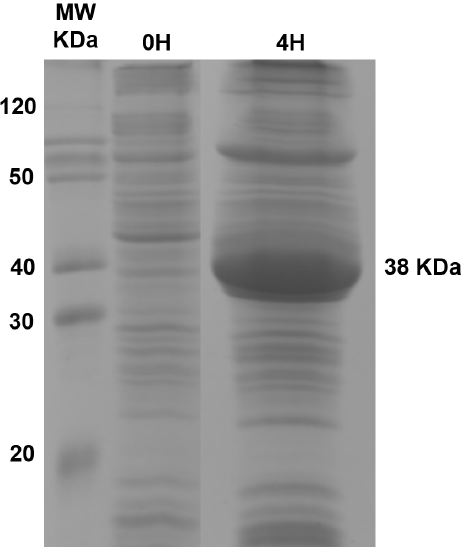
Figure 1 SDS-PAGE gel of the total protein concet of E. coli-CynD. The band at 38 kDa corresponds to CynD.
Enzymatic degradation of cyanide by E. coli-CynD
Industrial applications of microbes can utilize whole cells in either their growth or resting phase, or as permeabilized cells (Ramesh et al., 2016). We examined the activity of different types of cell preparations of E. coli-CynD to degrade cyanide (Table 1). Initial velocity results indicated that growing and resting cells are equally active, however, permeabilized cells are less active. Nevertheless, all cells completely depleted cyanide from a 5 mM solution within 10 minutes. In contrast, cyanide removal by untransformed E. coli cells was statistically equal to that caused by evaporation (0.42±0,7%). For the bioremediation of industrial cyanide wastewater, the use of a resting-cell process (Law et al, 2006) where the production of the biocatalysts through fermentation is separated from the biocatalytic process can overcome the cost associated with using complex media for biomass production. For all subsequent experiments reported here, resting (stationary) phase cell cultures were used.
Table 1 Effect of the cell resuspension solution over the activity of E. coli-CynD.
Cell type | Resuspension solution | Initial velocity (umol min"1 mg"1) | Cyanide removed (%) |
---|---|---|---|
Growing | LB broth | 2.44 ± 0,04 | 99.07 ± 0.8 |
Resting | 20 mM Tris Buffer (pH 8.0) | 2.78 ± 0,04 | 98.48 ± 0.7 |
Permeabilized | 20 mM Tris Buffer (pH 8.0), 5% Toluene, 1 mM EDTA | 1.72 ± 0,01 | 98.63 ± 1.3 |
Untransformed | 20 mM Tris Buffer (pH 8.0) | ND | 0.42 ± 0.7% |
E. coli-CynD activity at alkaline pH
HCN and CN- species exist in a pH-dependent equilibrium where the anion dominates at high pH. Thus, industrial cyanide solutions are maintained at pH 10 or above to avoid the dangerous volatilization of HCN (Johnson, 2015). The activity of resting E. coli-CynD cells at pH 8, 9 and 10 was examined (Figure 3). The alkaline pH degraded or inactivated the intracellular enzyme. Still, at pH 9.0 the enzyme retained 20% of the activity. This result contrast with the marked fall-off in activity at pH above 8.5 observed for the purified CynD enzyme (Jandhyala et al., 2005). Nevertheless, the residual activity of intracellular CynD was enough to achieve cyanide removal close to 100% at 20 minutes at pH 9.0 and 78% after 40 minutes at pH 10 (Data not shown).
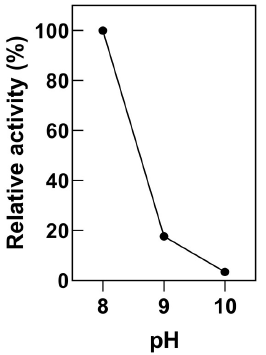
Figure 3 Activity of E. coli-CynD at alkaline pH Activity is calculated relative to the activity at pH 8.0. Data represent the mean ± sd of three independent experiments
Recently, protein engineering efforts have produced mutant CynD enzymes that retain activity at pH 9.5 (Crum et al., 2015; Crum et al., 2016). It is plausible that the use of whole cells overexpressing the more pH-resistant forms of CynD could provide a way to extend the pH tolerance of these enzymes to even more alkaline values.
Thermostability of E. coli-CynD
Industrial processes that involve cyanide solutions can operate at temperatures above 42 °C, which is a common occurrence in tropical countries. Purified recombinant wild-type CynD displays maximum activity between 37 °C and 42 °C (Jandhyala et al., 2005). However, it displays low thermal stability and activity is completely lost after a 5 hour incubation period at 42 °C (Crum et al., 2015; Crum et al., 2016). In contrast, intracellular CynD was more resistant to high temperature and it retained > 60% activity at 50 °C (Figure 4).
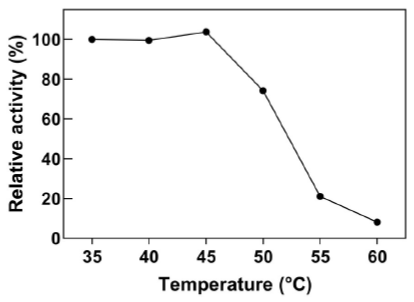
Figure 4 Optimal temperature range for E. coli-CynD. Relative activities were calculated with respect to the activity at 35°C
Thermal instability is a common feature of cyanide degrading enzymes such as CynD and cyanide hydratase (CHT) (Jandhyala et al., 2005). When incubated at 42 °C, purified wild-type CynD rapidly loses its cyanide-degrading activity (Crum et al., 2015; Crum et al., 2016), and at 55 °C, it becomes completely inactive in a matter of minutes (Jandhyala et al., 2005) . In contrast, intracellular CynD remains fully active at 42 °C for up to 72 h and retains 30% of its activity at 60 °C over the time course in which the purified enzyme becomes completely inactive (Figure 5). The thermostability of intracellular CynD even surmounts that of the most thermostable CynD chimera produced through protein engineering efforts ( Crum et al., 2015). The mutant enzyme formed by swapping the C-terminal sequence of CynD from B. pumilus with that of P. stutzeri AK61 displayed less than 20% residual activity after 42 h at 42 °C; whereas E. coli-CynD remained fully active within the same time (Figure 5). Similar patterns for the protection of enzyme activity are common when using whole cells to overexpress thermolabile enzymes. Furthermore, these approaches highlight the benefits of using whole cells for industrial applications in environments that are unfavorable for the activity of the enzyme (Wachtmeister & Rother, 2016).
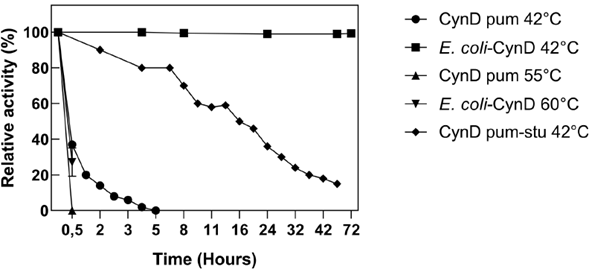
Figure 5 Thermostability of E. coli-CynD. For comparison, data for purified CynD (CynD pum) and the most thermostable chimera (CynD pum-stu) are shown (M.A. Crum et al., 2015; Mary A. Crum et al., 2016)
Heavy metal inhibition of E. coli-CynD
Purified CynD enzyme is strongly inhibited by Hg2+ and Pb2+ (D. M. Jandhyala et al., 2005). Analysis of the metal content of a solution obtained from a gold mine in Colombia revealed that Fe2+, Hg2+, and Ni2+ were abundant. Therefore, cyanide removal by E. coli-CynD was determined in separate solutions of these metals (Figure 6). In all cases, the metal concentrations tested were higher than those found in the solution obtained from the gold mine. The level of inhibition of E. coli-CynD was less pronounced than observed for the purified enzyme where cyanideremoval activity falls below 10% (Jandhyala et al., 2005).
Bioremediation of gold mining cyanide wastewaters
Gold mine derived cyanide wastewater is maintained at a very alkaline pH (>11). Additionally, it also contains high cyanide concentrations and variable concentrations of heavy metals and minerals. Therefore, the ability of E. coli-CynD to degrade free cyanide in wastewaters collected from a Colombian gold processing plant was tested (Figure 7). Results indicated that the intracellular CynD was completely inhibited when cells were added directly to the mine wastewater (Figure 7, Treatment A).
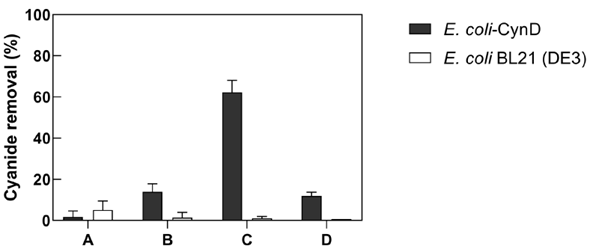
Figure 7 Ability of E. coli-CynD to remove free cyanide from gold mine wastewater. A. 1:10 dilution of E. coli-CynD cells to cyanide solution B. 1:10 dilution of E. coli-CynD cells to a 30-fold dilution of the cyanide solution. C. 3:10 dilution of E. coli-CynD cells to a 30-fold dilution of the cyanide solution. D. 1:2:10 dilution of E. coli-CynD cells to a 30-fold dilution of the cyanide solution.
Therefore, the wastewater solution was diluted 30-fold with water. The E. coli-CynD cells, however, could only degrade 14% of the free cyanide in that diluted solution (Figure 7, Treatment B). However, when the concentration of cells added increased by three-fold, cyanide degradation in the diluted solution was almost 70% (Figure 7, Treatment C). A possible explanation for the increase in cyanide-removal activity under the latter conditions is the potential interaction of cellular components with the inhibiting molecules present in the cyanide solution.
The results of treatment D (Figure 7) suggest that it is not any endogenous E. coli cellular component that prevents the inhibition, but rather that CynD itself is the molecular entity which chelates the inhibitors present in the wastewater solution. This hypothesis is supported by the fact that when untransformed E. coli cells are added together with E. coli-CynD cells, cyanide-removal activity only reached a level observed with cells expressing CynD. Similar results were obtained with E. coli-CynD cells and E. coli cells transformed with an "empty" plasmid.
A further explanation for the prevention of inhibition when higher concentrations of transformed cells are used is that, if heavy metals in the cyanide waste solution are the source of inhibition, a portion of the overex-pressed enzyme could bind to the heavy metal compounds and still leave enough "free" enzyme to exert catalysis of free cyanide. A conserved cysteine-glutamate -lysine triad characterizes enzymes of the nitrilase super family (Park et al., 2017). Therefore, the catalytic triad in CynD could act as metal chelator. The strong inhibition of the purified enzyme by Hg2+ and Pb2+ (Jandhyala et al., 2005) supports our hypothesis.
Our results, using E. coli-CynD demonstrated that by adjusting the ratio of live cells to dilutions of the cyanide-containing effluent, biodegradation of the free cyanide in the solution can be achieved. Moreover, these results encourage the use of a bioreactor based on E. coli-CynD cells. Cyanide effluents in this bioreactor could be recirculated, until legal cyanide limits for disposal are met. In the present study only free cyanide degradation was determined. Experiments designed to determine the degradation of total cyanide (WAD or SAD cyanide) by E. coli-CynD are in progress.
E. coli-CynD viability in gold mining cyanide wastewaters
Strict regulations exist for the use of recombinant microorganisms in open environments, such as gold processing plants. E. coli-CynD is a recombinant organism and concerns may exist about their fate in the environment. Therefore, the cellular viability of recombinant E. coli-CynD cells in the industrial cyanide waste solution was determined (Figure 8). Results indicated that permeabilized E. coli-CynD cells lose their viability after three days in the gold mine processing wastewater. The use of permeabilized cells, however, is undesirable due to the need to use organic solvents to produce them. Therefore, bioreactors utilizing immobilized E. coli-CynD on solid supports (Carmona & Panay, 2019) should be explored for the bioremediation of industrial cyanide wastes.
CONCLUSION
The use of whole E. coli cells overexpressing CynD presents an improvement over the thermostability of the purified CynD enzyme. The capacity of the intracellular enzyme to retain > 60% activity at 50 °C is a quality that can be exploited for the immobilization of the catalyst in thermogels like agarose. Also, improvement was observed in the pH resistance of the intracellular enzyme. Finally, our results indicated that E. coli-CynD is a viable alternative for the biodegradation of free cyanide in harsh industrial solutions like those derived from the mining industry.