Introduction
More than 60 years ago, McCormick proposed the usage of vitamin C (ascorbate) to limit the cancer metastasis process (McCormick, 1954, 1959). In the 1970s, Cameron and Pauling supported this concept, using high intravenous doses of vitamin C, followed by high oral doses, for advanced cancer patients (Cameron & Pauling, 1976, 1978). The reported anticancer effects of vitamin C were not unequivocally convincing, and the use of high-dose ascorbate in the clinical treatment of human tumors is controversial even up to now (Roa, etal., 2020; Bakalova, etal., 2020; Giansanti, etal., 2021; Zasowska-Nowak, et al., 2021). On the other hand, anticancer properties of ascorbate have been demonstrated in experiments with various cancer cell lines in vitro (Verrax, et al., 2006; Park, 2013; Ma, et al., 2017; Lv, et al., 2018; Semkova, et al., 2020) and in animals (Verrax, et al., 2006; Polireddy, et al., 2017; Xia, et al., 2017; Lv, et al., 2018) including aggressive tumor xenografts in mice (Chen, et al., 2008) and many other aggressive tumors (see for references Polireddy, et al., 2017).
Anti-metastatic and other beneficial effects of intravenous high-dose ascorbate in patients with advanced stage tumors were shown in preclinical trials (Polireddy, et al., 2017; Xia, et al., 2017; Bazzan, et al., 2018; Zhou, et al., 2020; Zasowska-Nowak, et al., 2021). It is very important to highlight that the high intravenous doses of ascorbate needed for anticancer effects allow plasma concentrations up to two orders of magnitude higher than concentrations achievable at the highest tolerated oral doses (Zasowska-Nowak, et al., 2021).
The mechanism of the ascorbate anticancer activity is not yet clear and appears to have multiple etiologies (Park, 2013; Ma, et al., 2017; Polireddy, et al., 2017; Lv, et al., 2018; Bakalova, et al., 2020). At least one of them might relate to the ascorbate oxidation in mitochondria as these organelles play a crucial role in switching cell energy metabolism from the preferentially aerobic oxidative phosphorylation in normal cells to the aerobic glycolysis observed in many malignant tissues, a process known as the Warburg type metabolism (Nakashima, etal., 1988; Lemeshko, 2002; Marín-Hernández, etal., 2006; Maldonado, et al., 2013). Previously, we explained such metabolic switching as a result of the metabolically-dependent generation of OMP by the VDAC-HK complexes (Lemeshko, 2014, 2015, 2017) functioning as a biological battery (Lemeshko, 2017, 2021). The generated OMP, positive in the mitochondrial intermembrane space (IMS), restricts the mitochondrial outer membrane (MOM) permeability to negatively charged metabolites, mainly for ATP (Rostovtseva & Colombini, 1997; Colombini, 2016).
Ascorbate is known to be oxidized very slowly in intact mitochondria, which allows assuming that MOM is impermeable to this anion (Lehninger, et al., 1954; Valenti, et al., 2014). In contrast, we have suggested that metabolically-dependent OMP of relatively high magnitude might be generated during ascorbate oxidation (Lemeshko, 2018, 2021) leading to the electrical closure of free VDACs in MOM and decreasing MOM permeability to this anion (negative feedback control). We also assumed (Lemeshko, 2021) that this ascorbate-dependent tendency to generate OMP (Figure 1A) negative in IMS might modulate the OMP generated in the mitochondria of cancer cells, controlling cell energy metabolism.
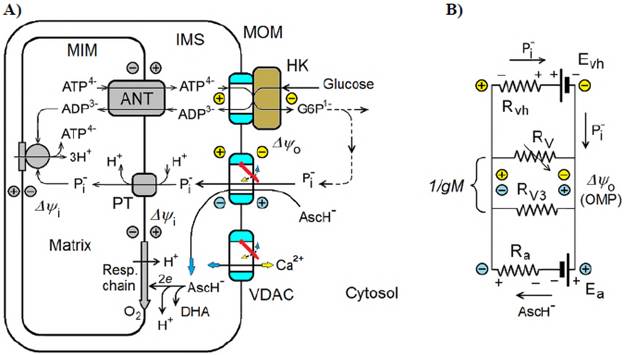
Figure 1 Modulation of the MOM permeability in cancer cells by the mitochondrial oxidation of ascorbate, AscH-. A. Ascorbate oxidation allows decreasing the magnitude of the positive OMP generated by the VDAC-HK complexes leading to the reopening of free VDACs in MOM, thus reprogramming the cancer type of cell energy metabolism and decreasing electrical, OMP-dependent Ca2+ extrusion from mitochondria. B. Equivalent electrical circuit of model A where the VDAC-HK complexes function as a biological battery (E hv ) with the internal resistance R vh maintaining the circulation of inorganic phosphate through MOM and generating the positive OMP. Steady-state transportation and oxidation of ascorbate in IMS have a tendency to generate the opposite, negative OMP, thus decreasing the magnitude of the final positive OMP (Δψ o) and increasing MOM permeability. MIM: Mitochondrial inner membrane; ANT: Adenine nucleotide translocator; PT: Inorganic phosphate transporter; DHA: Dehydroascorbate; E a: Ascorbate Nernstian potential; R V: Resistance of free VDACs in MOM (VDAC1 and VDAC2); R V3: Resistance of free VDAC3 in MOM; R a Internal resistance of the battery E a gM: MOM permeability
Here we used an essentially simplified computational model to evaluate the proposed hypothesis and demonstrate that the magnitude of ascorbatedependent OMP is sufficiently high to decrease significantly the positive OMP generated by the VDAC-HK complexes in mitochondria of cancer cells (Ay o in figure 1B), thus reprogramming the type of cell energy metabolism and decreasing cell death resistance. This mechanism may be modulated by many physiological factors influencing the VDAC-voltage sensitivity, VDAC-HK interactions, and VDAC permeability. All these aspects seem to be useful for developing new strategies for anticancer combination therapy.
Materials and methods
Model for the generation of the mitochondrial outer membrane potential by VDAC-hexokinase complexes
According to the model shown in figure 1A, some percentage of VDACs in MOM forms VDAC-HK complexes functioning as a biological battery (E vh in Figure 1B) (Lemeshko, 2017, 2018, 2021). Part of the battery voltage drops on the MOM (Ayo) and another part is applied to the internal resistance R vh of the battery (Figure 1B). R vh is inversely proportional to the fraction of VDACs bound to HK, N v. According to the model, 10% of all VDACs in MOM are represented by the low voltage-sensitive porin isoform VDAC3, A3, which does not bind to HK. The major fraction of free VDACs in MOM is represented by the voltage-sensitive VDAC isoforms, TV, such as VDAC1 and VDAC2. The quantity of all VDACs in MOM was normalized to 100:
where N 3 = 10 and N vh was taken for estimations equal to 4.0 or 3.6 relative units (r.u.).
The conductance of all MOM fractions of VDACs in their completely open states was also normalized to 100 r.u.:
where gV 0 is the conductance of the VDAC fraction N V at zero membrane potential, gH is the conductance of the fraction N vh of completely open VDACs, yet not restricted by the HK binding (numerically, gH = N vh). g3 = 10 r.u., assuming that the permeability of the VDAC3 fraction is completely voltage-insensitive, although the model also allows consideration of the redox-dependent modulation of the VDAC3 voltage sensitivity (De Pinto, et al., 2016).
The conductance gVH of the fraction N vh of VDACs bound to HK in MOM was considered to depend on the glucose concentration in the cytosol, [Gl]o, as (Lemeshko, 2017):
where the Michaelis-Menten constant KmG = 0.1 mM glucose. In other words, gVH also represents mitochondrial hexokinase activity in relative units considering 7Vh as the maximum HK activity. The MOM permeability gV through the fraction TV of free voltage-sensitive VDACs was considered to be equal for both the inorganic phosphate P i -1 and the ascorbate anion AscH-, and expressed as a bell-shaped function of OMP (Lemeshko, 2014, 2017):
where g C = 0.2 is taken as the relative VDAC permeability in the closed state and S is the voltage sensitivity parameter. The resulting normalized "VDAC's conductance-OMP" functions at the voltage sensitivity parameters S = 40 V-1 and S = 36 V-1 are shown in figure 2 (a and b, respectively).
The total MOM permeability, gM, for P i- 1 or the ascorbate anion AscH- was presented as:
The voltage generated by the VDAC-HK complexes (E vh in figure 1B) depends on the Gibbs free energy of the mitochondrial HK reaction (Lemeshko, 2014, 2017, 2021) as:
where ΔG o HK = -16.7 kJ/mol is the standard Gibbs free energy of the hexokinase reaction, R is the universal gas constant, F is the Faraday constant, and T = 310 K is the normal body temperature. [ADP], and [ATP]i. are IMS concentrations of ADP and ATP, respectively, [Gl]o and [G6P] o are the concentrations of glucose and glucose-6-phosphate, respectively, in the cytosol. For all estimations, it was taken that the ratio [ATP]i /[ADP]i . = 100 and [G6P]o = 0.1 mM.
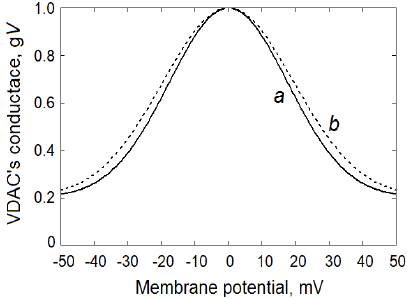
Figure 2 VDAC voltage-gating properties used in the computational model: a) at the VDAC voltaje sensitivity parameter S = 40 V-1; b) at S = 36 V-1 (equation 4)
In the absence of ascorbate (i.e., at E a = 0), the equivalent electrical circuit shown in figure 1B may be described according to Ohm's law as:
where I h is the hexokinase-mediated current (in dimensionless units).
According to equation 7, the OMP generated by the VDAC-HK complexes (Δψ o in figure 1B) may be presented as the voltage drop on the MOM resistance, 1/gM:
The units of OMP (equation 8), as well as of Evh (equations 6 and 7), come in volts. If a negative MOM potential f is generated by some mechanism simultaneously with the functioning of the VDAC-HK complexes, the resulting OMP may be presented as:
where f (of negative sign) is given in volts.
The system of equations 1-9 may be solved at given parameters g3, gH and f, calculating gV, gM and OMP as functions of glucose concentration in the cytosol.
Model of ascorbate-dependent generation of the outer membrane potential in intact mitochondria
The steady state rate of ascorbate oxidation in intact mitochondria, ν A, should be equal to the Nernst-Planck flux of this anion through MOM:
where [A] o and [A] i are the ascorbate concentrations in the cytosol and IMS, respectively, and OMPA is the MOM potential generated by the ascorbate flux across the membrane.
The rate vA of ascorbate oxidation by cytochrome c in IMS is presented by the Michaelis-Menten kinetics,
where V m is the maximum rate of oxidation in the same relative units (r.u.) as the hexo-kinase activity, gVH, the constant Km,A = 66 mM ascorbate (Yonetani, 1960), and is the concentration of ascorbate in IMS. For calculations, V m was taken at 0, 4 or 40 r.u. and N vh = 4 r.u. OMPA was estimated by solving the system of equations 1, 2, 4, 5, 10 and 11 as a function of ascorbate concentrations.
The software Mathcad Professional was used to perform all mathematical calculations.
Results
The MOM VDAC-HK complexes function as a biological battery (Lemeshko, 2017, 2018, 2021) due to an active movement of the inorganic phosphate anion through MOM (Figure 1A) from ATP4- in IMS to glucose in the cytosol forming glucose-6-phosphate. This movement depends on the free energy of the hexokinase reaction. Inorganic phosphate then returns into IMS, thus closing the electrical circuit to recover ATP in the mitochondrial matrix. In this case, OMP generation may be presented as a part of Evh, i.e., as the voltage drop on the equivalent resistances 1/gM of MOM (Lemeshko, 2014, 2021) (Figure 1B).
According to our estimations using the computational model, the magnitude of OMP, positive in IMS, depends on the concentration of glucose (Figure 3, curve a). With a 10 % decrease in the quantity of VDAC-HK complexes in MOM, from 4 r.u. (Figure 3A, curve α) to 3.6 r.u. of all VDACs in MOM (Figure 3B, curve α), the critical glucose concentration at which OMP begins to increase sharply shifted from 2 mM (at 4 r.u.) to 6 mM (at 3.6 r.u.), approximately.
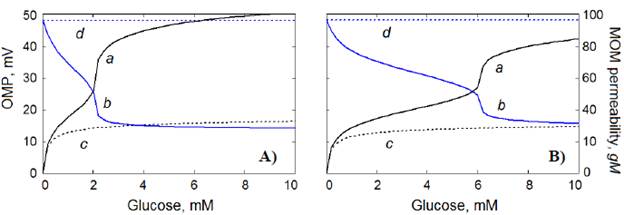
Figure 3 Generation of OMP (α, c) by the VDAC-HK complexes in mitochondria and the MOM permeability to inorganic phosphate and ascorbate (b, d) as functions of glucose concentration. The fraction N vh of the VDAC-HK complexes - 4.0 r.u. (A) or 3.6 r.u. (B). The VDAC voltage sensitivity parameter S = 40 V-1 (a, b) or S = 0 V-1 (c, d) (equation 4). Calculations were performed according to section 2.1.
The model also demonstrated a remarkable OMP-dependent decrease in the MOM permeability, gM (Figure 3, curve b). In contrast, at the zero VDAC voltage sensitivity parameter, S = 0 V1 (equation 4), the generated OMP has relatively low magnitudes (Figure 3, curve c) without its somewhat essential influence on the MOM permeability (Figure 3, curve d).
The model also demonstrated that a negative MOM potential, f generated by any mechanism significantly influences the resulting OMP (equation 9) generated during the simultaneous functioning of the VDAC-HK complexes. The potential f of the magnitudes higher than f = -6.5 mV for the case of 4.0 r.u. of the VDAC-HK complexes (Figure 4A) and the potential higher than f = -2.0 mV for the case of 3.6 r.u. of the VDAC-HK complexes (Figure 4C), completely prevent a sharp glucose-dependent increase in OMP, at least in the range up to 10 mM glucose concentration, thus allowing higher MOM permeability, gM (Figure 4, B and D, respectively).
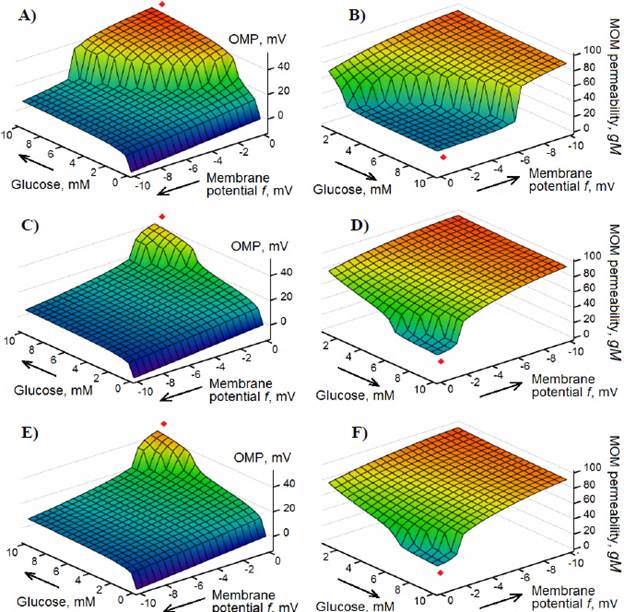
Figure 4 Influence of the negative membrane potential f generated by any mechanism simultaneously with the generation of positive OMP by the VDAC-HK complexes in mitochondria on the resulting OMP (equation 9) (A, C, E) and the MOM permeability gM (equation 4) (B, D, F). The fraction N vh of the VDAC-HK complexes - 4.0 r.u. (A, B) or 3.6 r.u. (C, D) at the VDAC voltage sensitivity parameter S = 40 V-1. E, F: N vh = 4.0 r.u. and S = 36 V-1
Similar data to the case of a 10 % decrease in the quantity of the VDAC-HK complexes in MOM (Figure 4, C and D) were also obtained by a 10 % decrease in the VDAC voltage sensitivity parameter, from S = 40 V-1 to S = 36 V-1 (Figure 4, E and F). Besides, a simultaneous 10% decrease in the quantity of the VDAC-HK complexes and the VDAC voltage sensitivity completely prevented any sharp glucose-dependent increase in OMP (data not shown).
In other words, the data presented in figure 4 demonstrate that a decrease in the quantity of hexokinase attached to mitochondria and/or a decrease in the voltage sensitivity of the VDAC gating mechanism, strongly influence glucose-dependent VDAC-HK-mediated regulation of the MOM permeability.
To evaluate the magnitude of the MOM potential generated only under ascorbate oxidation in intact mitochondria, OMPA, the estimations were performed assuming completely silenced VDAC-HK complexes in MOM (N vh = 4 r.u.), as it would be at zero concentration of glucose. The model demonstrated the generation of relatively high OMPA negative in IMS at different maximum rates, V m of ascorbate oxidation by cytochrome c in IMS and at given concentrations of ascorbate ([A]o and [A]i) (Figure 5).
First of all, if we assume the rate V m = 0, i.e. the thermodynamic equilibrium, we have Nernstian potential at given values of [A]o and [A]i. (Figure 5A). At steady-state with V m = 4 r.u. and the VDAC voltage sensitivity parameter S = 40 V-1, the model demonstrated OMPA of lower magnitudes (Figure 5B) than Nernstian potentials, as well as a more significant decrease in OMPA at higher V m , for example at V m = 40 r.u. (Figure 5C). A decrease in OMPA at steady states is a result of the voltage drop (part of the Nernstian potential) on the internal resistance R of the ascorbate battery E a (Figure 1B). In the case of V m = 40 r.u., if we additionally decrease the voltage sensitivity parameter from S = 40 V-1 to S = 0 V-1, the model demonstrates significantly increased magnitudes of OMPA, in the same range of given concentrations of ascorbate (Figure 5D) due to a lower voltage drop on the resistance R a (Figure 1B).
In general, the model demonstrates that the membrane potential of relatively small magnitudes, negative in IMS (f in figure 4) and generated by any mechanism, might modulate the positive OMP generated by the VDAC-HK complexes and the MOM permeability. Additionally, it was clear that the ascorbate oxidation in intact mitochondria functions is one of these mechanisms and it has the tendency to generate the negative membrane potential.
Discussion
As a natural product, ascorbate is of great interest for its possible clinical use in tumor treatments, at least in combination with other methods of chemotherapy. Besides, it does not demonstrate noticeable toxicity against normal tissues, even at high doses, having, instead, various positive effects for the treatment of advanced-stage cancer (Polireddy, et al., 2017; Xia, et al., 2017; Bazzan, et al., 2018; Zhou, et al., 2020; Zasowska-Nowak, et al., 2021). According to the literature data (Park, 2013; Ma, et al., 2017; Polireddy, et al., 2017; Lv, et al., 2018; Bakalova, et al., 2020), there seem to be multiple mechanisms of the ascorbate anticancer activity that are not entirely understood. It means that many factors might modulate its efficiency in the treatment of malignant tissues (Noto, et al., 1989; Verrax, et al., 2006; Gogvadze, et al., 2008; González, et al., 2012; van Gorkom, et al., 2019). Here we propose a new mechanism of ascorbate anticancer activity focusing our attention on the great difference in the energy metabolism of cancer versus normal cells (Nakashima, et al., 1988; Lemeshko, 2002; Marín-Hernández, et al., 2006; Gogvadze, et al., 2008; González, et al., 2012; Maldonado, et al., 2013).
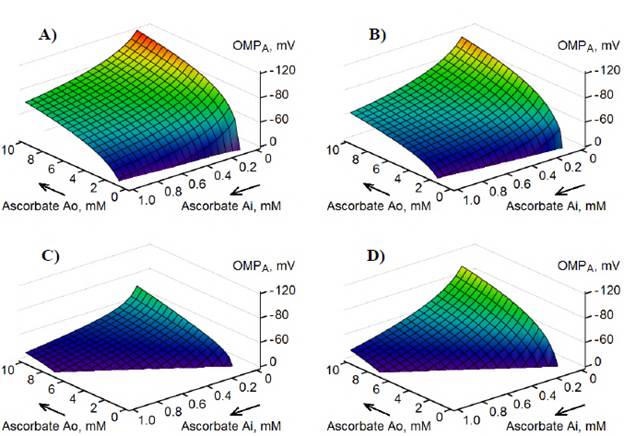
Figure 5 Generation of OMPA during ascorbate oxidation in intact mitochondria at V m = 0 r.u. (A), Vm = 4 r.u. (B), Vm = 40 r.u. (C, D), S = 40 V -1 (A-C), or S = 0 V -1 (D). Estimations were performed at N vh . = 4 r.u. V m : Maximum rate of ascorbate oxidation in IMS.
We had already explained the Warburg type metabolism of cancer cells as a result of the electrical suppression of mitochondria by the metabolically-dependent OMP generated by the VDAC-HK complexes in MOM (Lemeshko, 2002, 2014, 2015, 2021). According to the computational model presented in this work (Figure 1), the critical glucose concentration needed for the generation of relatively high-magnitude OMP strongly depends on the quantity of these complexes in MOM (Figure 3). Our estimations demonstrate that the superposition of a relatively small negative OMP (f) generated by any mechanism and the positive OMP generated by the VDAC-HK complexes strongly decreases the magnitude of the resulting OMP. This OMP decrease leads to the reopening of the electrically closed free VDACs in MOM, thus increasing MOM permeability (Figure 4).
The model demonstrated that the effect of the mentioned negative potential f on the MOM permeability strongly depends on the quantity of the VDAC-HK complexes. A mere 10% decrease in the quantity of the VDAC-HK leads to a significant decrease in the glucose-dependent OMP (Figure 4C), thus increasing MOM permeability (Figure 4D). In this respect, many factors that can prevent the formation of the VDAC-HK complexes in MOM, or induce their dissociation, have been reported to demonstrate pro-apoptotic and anticancer activities (Galluzzi, et al., 2008; Camara, et al., 2017; Magri, et al., 2018; Shteinfer-Kuzmine, et al., 2018; Ciscato, et al., 2020). The mechanism of such pro-apoptotic and anticancer activities might involve the mentioned reopening of the electrically closed VDACs in the mitochondria of cancer cells.
The model also demonstrated that the modulation by a negative membrane potential f of OMP generated by the VDAC-HK complexes strongly depends on VDAC voltage sensitivity allowing the reopening of the electrically closed VDACs. A decrease of only 10% in the voltage sensitivity parameter S leads to a strong decrease in OMP, thus increasing MOM permeability (Figure 4, E and F). In this respect, VDAC voltage sensitivity may be essentially modulated by many factors (Rostovtseva, et al., 2020, 2021) including cytosolic NADH and NADPH (Lee & Colombini, 1996).
Some cytosolic proteins, such as tubulin and alpha-synuclein, have been demonstrated to cork up free VDACs in MOM assuming this process is strongly OMP-dependent (Rostovtseva, etal., 2021). VDAC corking up, in turn, should also modulate OMP generation (Lemeshko, 2017, 2018, 2021).
As we assumed earlier (Lemeshko, 2018, 2021), ascorbate oxidation in intact mitochondria might generate OMP negative in IMS (Figure 1) thus explaining a very low rate of its oxidation in these organelles (Lemeshko, et al., 2006; Valenti, et al., 2014). The ascorbate anion is almost three times smaller than ATP4-, although ATP4- is permeable through the open VDAC (Rostovtseva & Colombini, 1997; Colombini, 2016; Rostovtseva, et al., 2021). That is why there are no reasons to believe that MOM is impermeable to the ascorbate anion as assumed earlier (Lehninger, et al., 1954; Valenti et al., 2014). To the best of our knowledge, no experimental data regarding the VDAC permeability to ascorbate has been registered yet.
On the other hand, if ascorbate oxidation in mitochondria has the tendency to generate OMP negative in IMS (Figure 5), the anticancer effect of high-dose ascorbate might relate to the modulation of the positive OMP generated by the VDAC-HK complexes. The effect might be similar to that shown in figure 4 for a negative potential f generated by any mechanism. Moreover, the positive VDAC-HK-dependent OMP might strongly increase ascorbate oxidation in the mitochondria of cancer cells by increasing steady-state-concentrated ascorbate in IMS. This conclusion is consistent with the experimental observations of vitamin C deficiency in cancer patients and malignant tissues (Van Gorkom, et al., 2019; Blaszczak, et al., 2019).
It has been reported that electrically-closed VDACs strongly restrict the release of superoxide anions from mitochondria (Han, et al., 2003) meaning that if the VDAC-HK complexes in MOM generate OMP, there should be a relatively high concentration of superoxide anions maintained in the IMS of the mitochondria of cancer cells, as well as of ascorbyl-free radical anions due both to the electrical closure of free VDACs in MOM and to the generated OMP positive in IMS.
Interestingly, the protective, anti-apoptotic effect of mitochondrial HK in cancer cells has been considered to be a result not only of the VDAC's binding to MOM (two orders of magnitude higher than in normal cells) but also of the functioning of HK bound to the mitochondria (Sun, et al., 2008), both important for the metabolically-dependent generation of OMP (Lemeshko, 2014, 2021). For example, the positive VDAC-HK-dependent OMP of 30 mV should maintain Ca2+ concentration in IMS one order of magnitude less than in the cytosol thus strongly preventing the Ca2+ overload of the mitochondria (Figure 1A). This is consistent with the fact that malignant tissues are highly resistant to cell death (Han, et al., 2003; Gogvadze, et al., 2008; Sun, et al., 2008). The positive OMP generated by the VDAC-HK complexes may be decreased by applying the additional negative potential (f) (Figure 4) seemingly generated during ascorbate oxidation in the mitochondria of cancer cells (Figure 5). It should also facilitate Ca2+ entrance into IMS from the cytosol (Figure 1A) finally leading to Ca2+ overload of the mitochondrial matrix, even though the permeability to Ca2+ ions of VDAC in its electrically closed state is higher than in the completely open state (Tan & Colombini, 2007).
One of the main mechanisms suggested to explain ascorbate's anti-cancer properties is the ascorbate-induced ROS overproduction in cancer cells (Verrax, et al., 2006; Ma, et al., 2017; Xia, et al., 2017; Lv, et al., 2018; Semkova, et al., 2020; Zhou, et al., 2020) including ascorbyl radical generation that facilitates external NADH oxidation in mitochondria and changes the cytosolic NADH/NAD+ redox state (Bakalova, et al., 2020). Extremely high production of mitochondrial superoxide in cancer cells during ascorbate oxidation facilitated by menadione has also been reported (Semkova, et al., 2020). Increased ROS production in combination with Ca2+ overload of mitochondria should increase the probability of Ca2+-ROS induced mitochondrial permeability transition (Grimm & Brdiczka, 2007; Bernardi, 2013; Lemeshko, 2018, 2021). Taking into account ROS-dependent mechanisms of high-dose ascorbate toxicity for malignant tissues (Kang, et al., 2003; Carosio, et al., 2007; González, et al., 2010; Xia, et al., 2017; Zhou, et al., 2020), the possibility of generating the negative membrane potential during ascorbate oxidation (OMPA in figure 5) and its influence of the positive OMP generated by the VDAC-HK complexes in the mitochondria of cancer cells (Figure 4), the main mechanism of ascorbate anticancer activity seems to be combined OMP-Ca2+-ROS-dependent.
The anti-cancer activity of ascorbate has been reported in the treatment of many aggressive tumors, such as pancreatic cancer, glioblastoma, ovarian cancer, prostate cancer, hepatoma, colon cancer, sarcoma, leukemia, mesothelioma, breast cancer, and neuroblas-toma (see for references Polireddy, et al., 2017). Interestingly, activities of the respiratory chain complexes in glioblastoma (WHO grade IV), for example, are significantly decreased in comparison with a normal brain, especially of complexes III (by 81%) and IV (by 84%) (Feichtinger, et al., 2014). According to these data, the concentration of reduced cytochrome c in brain tumors and breast cancers is significantly higher than in normal human tissues (Abramczyk, et al., 2021). The mentioned decrease in the respiratory chain complexes in the mitochondria of cancer cells might be an adaptive consequence of the Warburg-type metabolism, explained earlier as a result of electrical suppression of mitochondria by OMP generated by the abundant VDAC-HK complexes (Lemeshko, 2015, 2021).
In this respect, ascorbate is known to be oxidized in mitochondria with the participation of the cytochrome c and complex IV of the respiratory chain. The parameter V m used in the model we present here (Figure 5) directly relates to complex IV and cytochrome c concentrations in IMS. The suggested model of the anti-cancer activity of ascorbate may be further developed in the future taking into account the mentioned changes in the respiratory chain, as well as the mutual interactions of the steady-state fluxes of inorganic phosphate and ascorbate across MOM.
It is well known that the redox mediators, such as menadione, PMS, TMPD, etc., strongly increase the rate of reduction of free cytochrome c by ascorbate, as well as the ascorbate oxidation in intact mitochondria (Sanadi, 1964, Hill & Nicholls, 1980; Nicholls, et al., 1980; Valenti, et al., 2014). This may explain the reported synergistic anti-cancer effects of ascorbate-menadione (Noto, et al., 1989; Gilloteaux, et al., 2006; Verrax, et al., 2006; Semkova, et al., 2020) and of the ascorbate use together with other redox mediators (Tomasetti, et al., 2015; Hua, et al., 2019).
In general, the results of the computational analysis of the suggested mechanism of ascorbate anti-cancer activity show that ascorbate oxidation in mitochondria has a tendency to generate negative OMP. This might modulate OMP generated by the VDAC-HK complexes in cancer cells leading to the reprogramming of the cell energy metabolism. This mechanism seems to be useful for developing novel and/or improving existing strategies of anti-cancer combination therapy (Ngo, et al., 2019; Semkova, et al., 2020), as well as for explaining in more detail various experimental phenomena in this respect.
Conclusion
Despite the long controversial history of ascorbate's anti-cancer properties, the mechanism of its selective toxicity at high doses for malignant tissues remains unclear and it seems to involve multiple processes and targets. Among possible targets underlying ascorbate anticancer effects, mitochondria might play a crucial role (Gogvadze, etal., 2008; González, et al., 2010, 2012; Hua, et al., 2019; Semkova, et al., 2020). In the case of fast-growing tumor cells, the quantity of hexokinase attached to MOM is up to two orders of magnitude higher than in normal cells (Marín-Hernández, et al., 2006). This allowed us to consider the VDAC-HK complexes in MOM as a biological battery (Lemeshko, 2017, 2018, 2021), generating high-magnitude OMP (positive in IMS) and switching cell energy metabolism to the Warburg type due to an electrical suppression of mitochondria (Lemeshko, 2015, 2021). On the other hand, the rate of ascorbate oxidation in isolated intact mitochondria of normal tissues is known to be extremely low, which seems to be caused by the generation of the negative OMP. The simplified computational model presented here demonstrated that a negative OMP of even low magnitudes generated by any mechanism might essentially decrease the magnitude of the positive OMP generated by the VDAC-HK complexes in cancer cells (Figure 1A) reprogramming the cell energy mechanism. This should also lead to the Ca2+-ROS mitochondrial permeability transition, the cytochrome c release from mitochondria, and cell death. Besides, since the suggested primary mechanism of selective toxicity of ascorbate for cancer cells strongly depends on the presence of redox mediators and on factors influencing the VDAC-HK interactions, VDAC's voltage sensitivity, and VDAC's corking up factors (Lemeshko, 2017), the suggested mechanism might be useful for the development of new experimental approaches and novel anti-cancer combination therapies.