This work es within the framework of the PIP Multi-year Research Project No. 11220150 100570CO entitled Social Technologies in Urban Habitats with Impoverished Populations. This work has been funded by the National Scientific and Technical Research Council (CONICET) and by the Architecture, Design and Urban Planning Department of the University of Buenos Aires, in conjunction with the Optimized Wattle and Daub Technology Research and Development Project for Housing in Colder Arid and Semi-Arid Argentinian Townships, also financed by CONICET.
In order to integrate exterior environments with architectural works, an increasing number of construction professionals conduct housing and urban projects that incorporate sustainability. Sustainability consists of adjusting human environments to meet a limiting factor: the environment's ability to meet human demands so that its natural resources are not irreversibly degraded (Alavedra, Domínguez, Gonzalo & Serra, 1997, p. 42 ).
Among industrial activities, construction, together with its associated industries, is the largest consumer of natural resources like wood, minerals, water and energy. Similarly, once constructed, buildings continue to be a direct cause of pollution due to the emissions they generate, thus impacting the regional environment by consuming energy and water for regular operations (Alavedra et al., 1997, p. 42).
The manufacturing stages for construction materials and their byproducts generally result in a high environmental impact. This impact starts with the extraction of natural resources to be used in the manufacturing process and continues with the energy consumed at each phase of the process. As a result, emissions enter the atmosphere as pollutants that can be corrosive and highly toxic. This process is repeated both in the operation and in the use of a building, until the materials are finally reduced to essential parts that will be recycled or reused in a new construction.
Sustainable construction criteria guide the pro-duction of buildings with decreased industrialized materials, thus avoiding, whenever possible, the use of materials that end their life cycle as dangerous waste or whose essential components are difficult to break down. The main environmental impact of construction materials include: energy consumption, solid waste, contributions to greenhouse effects, damage to the ozone layer and other factors of environmental pollution (Cáseres, 1996, pp. 7-8 ,; Wassouf, 2014).
Soil is assumed to be the oldest construction material that humanity has used, and it currently represents a solution to the demand for low-cost housing (Vega, Andrés, Guerra, Morán, Aguado & Llamas, 2011, p. 3021). Even today, 30% of the world's population lives in earth shelters (Freire & Tinoco, 2015, p. 18). This alternative has numerous justifications, including: the high availability of this raw material in nature, its reduced pollution and the low CO2 emissions during manufacture and transport stages (Piattoni, Quagliarini & Lenci, 2011, p. 2067), as well as generating zero waste, both at the construction and demolition stages; likewise, one of its most valuable properties is its thermal response, which is essential for comfort and for reducing the use of auxiliary heating or cooling systems throughout the life cycle of a building.
The most common construction systems with earth are adobe, wattle and daub, rammed earth and CEB. In these natural construction systems, most of the energy involved in production comes from the sun because they are dried in the open air, under the sun, without the need to resort to oven drying, as in fired brick. This reduces the consumption of non-renewable energy and the corresponding emissions.
An important characteristic of the proper functioning and amenity of a home environment is a comfortable design for its occupants. In this sense, the materials used in the building envelope are fundamental because they integrate the elements that separate the interior environment from the exterior. The choice of these elements depends on different factors, such as the technology that can be used, its structural response, its life cycle, and its aesthetics.
Given that earthen materials have a heterogeneous behavior, they cannot be typified to achieve a homogeneous response the way one can with concrete, for example. In the case of soil, its behavior will depend on the composition of each soil sample and each site (Minke, 2005, p. 16). Thus, construction elements made from different soils have different thermal, mechanical and physical responses.
The present work provides a compilation of data contributed by research projects and guide-lines regarding variables in thermal behavior and the mechanical and structural resistance of earthen construction materials. Some of these values are the result of experimental trials conducted in accredited institutions around the world. These refer to the properties of different construction technologies with soil, such as adobe, rammed earth, wattle and daub and CEB, among others. Some of the materials used in traditional construction have been taken as a point of reference, such as common fired brick, hollow ceramic brick and concrete. Based on this analysis, some considerations emerge that help determine the most important characteristics of earthen construction materials and the relationship between them.
Methodology
A comparative analysis method was used for both thermal properties and mechanical strength. To this end, a bibliography was com-piled containing extant publications by several authors, along with the data from regulatory guidelines and the experimental data obtained by the authors of the present work. First, the thermal and mechanical properties were defined for evaluation. Then, comparative tables were created for visualizing the thermal properties and densities that various authors obtained for each natural construction system (e.g. adobe, rammed earth, CEB and wattle and daub) as well as for the systems used in traditional construction (e.g. solid brick, hollow ceramic brick and cast-in-situ concrete).
To meet the minimum level of thermal comfort according to the bioenvironmental area, the mud-construction wall thicknesses analyzed reflect the maximum thermal transmittance values permissible for walls in Argentina according to the IRAM Standard.
Finally, the same comparative procedure was carried out for the mechanical resistances of both earthen and traditional construction systems. The vales under comparison were obtained by each author or each regulatory standard and are in reference to the material's resistance to cutting, bending and simple compression.
The conclusion reflects an analysis of the comparative thermal and mechanical resistance derived from the literature review, as well as the authors' own reflections on the conditions for a potential development of earthen construction in Argentina.
Results
Thermal and Mechanical Characteristics of the Materials
Below are the definitions that link each measured property with forces or energy flows that produce the values recorded in each corresponding table.
Thermal Properties
Thermal properties refers to the greater or lesser capacity to transmit heat or accumulate it, thus determining the thermal inertia of a construction. In reference to the material itself, these capacities can be defined as: density, specific heat and thermal conductivity. In reference to construction elements, such as horizontal enclosures (ceilings), transparent vertical enclosures (fixed frames, Windows and shutters) and opaque vertical enclosures (walls and doors), these capacities are defined as: thermal transmittance, heat capacity, thermal inertia and delay. The meaning of these thermal properties is pro-vided in the following sections.
Thermal Properties in Reference to Materials
Density (kg / m3): is the mass per volumetric unit of a body. The greater or lesser density of a construction material influences its insulating capacity, as will be analyzed later. It should be noted that density also affects mechanical properties.
Thermal conductivity X [W/mK]: is the amount of heat that is transmitted in one direction, per unit of time and surface area, when the temperature gradient in that direction is uniform.
Thermal Properties in Reference to Construction Elements
Thermal transmittance K [W/m2K]: is the amount of heat transmitted by an enclosure in steady state, per square meter of surface (perpendicular to heat flow), per unit of time and per temperature gradient unit between interior and exterior environments.
Thermal inertia: is the capacity that the mass of the materials has for absorbing and accumulating heat during daylight hours, which is then released in order to condition the interior environment (generally, at night). This helps to achieve better thermal comfort by reducing indoor temperature variations compared to outdoor temperatures. The energy transfer process is not instantaneous. There is a delay in time for heat transfer by conduction from one side of the wall to the other, known as thermal inertia. Figure 1 represents the concepts of thermal lag, the time elapsed while the heat absorbed by the wall reaches the opposite side, and damping, the difference in energy between the exposed face of the wall and interior (Gutierrez & Gallegos, 2015, p. 61).
Mechanical Properties
Mechanical properties refer to the most important variables for construction materials or technology. These properties are: simple compressive strength, tensile strength and shear resistance. Simple compressive strength is understood as the ability of materials to resist crushing loads before breaking. In the case of tensile strength, this is the ability of materials to withstand loads that tend to pull it before reaching breakage. Shear strength refers to the ability to withstand shearing loads. These resistances have the same expression; what changes in each case is the force that is imposed on the element (Cieck, 2005, p. 136).
Once the concepts of thermal and mechanical properties had been defined, the comparative analysis of each one was carried out with respect to the materials used both in earthen construction and in traditional construction, according to the results published by different authors.
Densities and Thermal Properties of Some Materials and Construction Elements used in Industrial and Earthen Construction
Table 1 shows the density, thermal conductivity and thermal transmittance of different soil mixtures and some earthen construction elements such as adobe, straw-mud mixture, solid mud, CEB and wattle and daub. Table 2 includes the corresponding values of density, thermal conductivity, thickness and thermal transmittance for walls of common fired brick, hollow ceramic brick and cast-in-situ concrete.
Table 1 Thermal properties of some earthen materials and construction elements of varied thickness, according to various authors
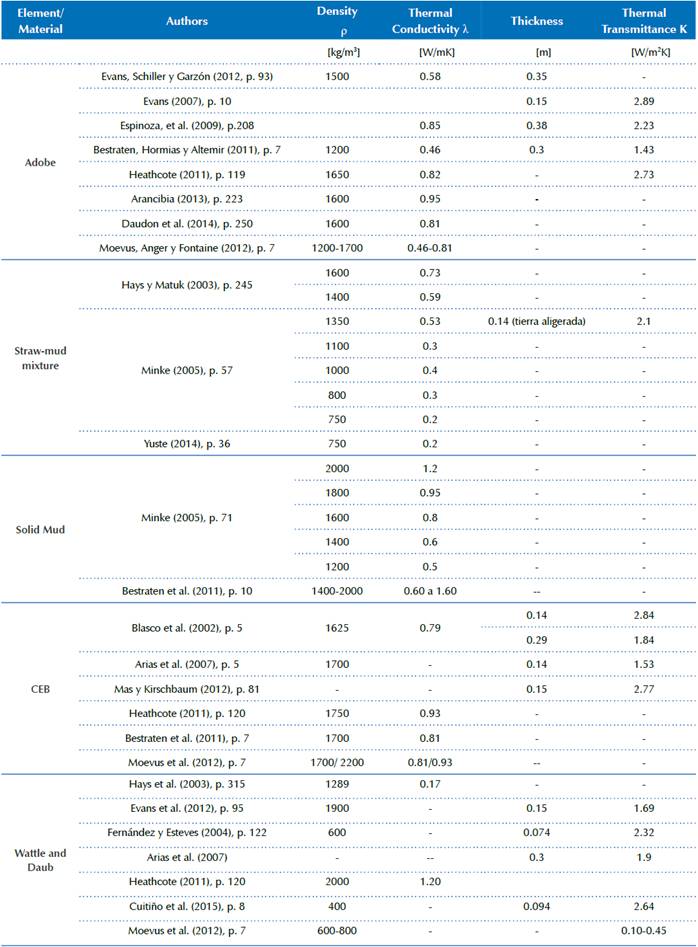
Source: author’s elaboration (2019).
Table 2 Thermal properties of some traditional materials and construction elements of varied thickness, according to various authors
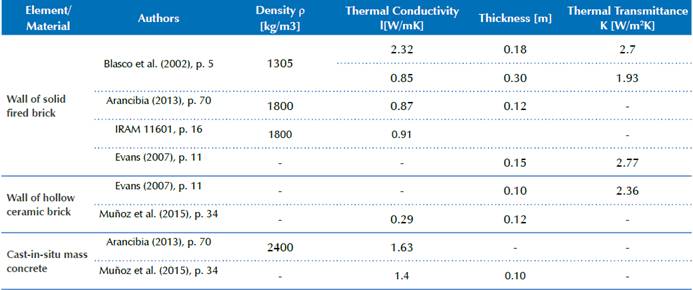
Source: Autor´s elaboration (2018).
Walls built with soil have density values that vary between 750 kg/m3, for straw-mud mixture, and 2000 kg/m3, for solid mud. In comparison, densities for industrialized materials can range between 1300 kg/m3, for common solid brick, and 2400 kg/m3, for cast-in-situ concrete.
Sourced from several authors, the thermal transmittance and thermal conductivity values that correspond to the density values of these materials are also provided. There are some differences between the thermal conductivity of the earthen constructions and the conventional ones. In the first case, it is based on values of 0.30 W/mK for straw-mud mixture, 0.95 W/mK for adobe, and 1.60 W/mK for solid mud, with variable thicknesses of 0.074 m for wattle and daub to 0.35 m for adobe.
In the second case, values range from 0.29 W/ mK, for hollow brick, up to 2.32 W/mK for solid brick, with thicknesses of 0.18m.
Figure 2 shows thermal conductivity values, in logarithmic scale, of the materials commonly used in traditional construction. Expanded polystyrene shows the lowest thermal conductivity, and copper shows the highest thermal conductivity. Figure 2 also plots the range of conductivity for earthen building systems, which vary between 0.46 W/mK and 1.00 W/mK, illustrating the low variation in thermal conductivity for earthen construction compared to materials commonly used in traditional construction.
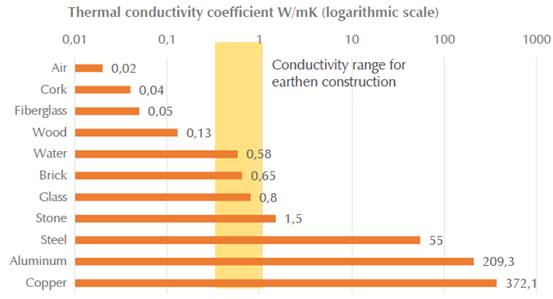
Source: author’s elaboration, based on edison (2018)
Figure 2 Comparison of thermal conductivity in construction materials in w/ mk (logarithmic scale)
An analysis of the relationship between material density and thermal conductivity (Figure 3) shows that materials with low densities have low values of thermal conductivity. This is because they have lower compaction and more voids, which results in a lighter and more insulating material compared to a denser and more compact material. This dynamic can be seen in the case of lightweight and cellular concrete, where the higher the density, the greater the conductivity. In the case of adobe and compacted soil, the density is relatively constant, so there is little variability in conductivity; however, in the case of light-weight soil and wattle and daub, the densities are low, due to the presence of more air and the manual construction technique used for raising the walls (Table 1).
Another aspect, analyzed by several authors, is the thermal lag of different construction systems. Table 3 shows the comparative thermal lag of an adobe wall versus a compacted soil wall, located in the Bioenvironmental Zone IIIb, with minimal thicknesses-according to IRAM Standard 11.605 (IRAM 11605, 1996, p. 16)-of 25 cm and 35 cm, respectively. These provide a thermal delay of 8.4 hours and 11.4 hours, both with a similar thermal transmittance. Neither case poses a risk of superficial or interstitial condensation.
Table 3 Thermal lag of an adobe wall compared to a compacted earth wall
Material | Adobe | Compacted soil |
---|---|---|
Minimum thickness, iram standard 11.605,c (1996) | 25 cm | 35 cm |
Thermal transmittance | 1.71 w/m2k | 1.79 w/m2k |
Thermal lag | 8.4 hours | 11.4 hours |
Admittance | 4.3 w/m2k | 4.9 w/m2k |
Superficial condensation (iram 11.625, 2000) | No | No |
Interstitial condensation (iram 11.625, 2000) | No | No |
Source: evans (2004, p. 15).
Similarly, the adobe thermal response is compared to traditional materials, such as concrete, brick and stone (Figure 4). It can be seen that in all materials there is a linear interrelation between the thickness of the wall and the thermal delay, where adobe occurs as an intermediate point between concrete and brick. If a more rigorous study were to be conducted, it should be conducted with the common brick wall thickness of 0.20 m, which carries a thermal lag of 6 hours. For concrete walls of the same thickness, the lag is 5 hours, but in adobe, walls are typically built with a thickness of 0.30 m, which results in a lag of 9 hours; that is, if the maximum peak of outside temperature occurs at noon, all the absorbed energy will be delivered to the indoor environment by 9 pm, which is when it is most necessary to achieve comfort. Omitting losses from the external surface of the element to the outside air, solar absorption of the outer surface is considered uniform, which indicates a constant value for all cases.
Analysis of the Maximum Admissible Values for Thermal Transmittance of Walls in Argentina
In order to optimize the vertical enclosures of a house, the IRAM 11.603 (2012) and IRAM 11.605 (1996) standards were used to determine the maximum admissible value Kmax A DM of thermal transmittance K in walls for each bioenvironmental zone in Argentina (Figure 5).
Afterwards, considering thermal transmittance as indicated in Tables 1 and 2, the enclosure is checked against the different bioclimatic zones according to the projected exterior temperature (in winter) of the capitals of each province. IRAM 11.605 indicates 3 levels of hygrothermal comfort: Level A: Recommended; Level B: Medium; and Level C: Minimum. These are partially defined by the non-existence of surface condensation when indoor air temperatures are maintained at certain values, in accordance with IRAM Standard 11.625. The present study was conducted under guidelines for Level C: a temperature of 182 C and a difference of up to 4° C between the model interior temperature and the interior surface temperature of the enclosure.
Table 4 shows the KMAX ADM values for cities in each province of Argentina, depending on the model exterior temperature (TED) according to the values of IRAM Standard 11.603.
Table 4 Maximum admissible thermal transmittance kmax adm values for each province in Argentina
Province | Model ext. Temp. (winter) | Bioenvironmental Zone | K max adm Values for winter | K max adm Values for summer |
---|---|---|---|---|
Figure 4 | Minimum level (c) | Minimum level (c) | ||
Buenos aires | 3.10 | llla | 1.85 | 2.0 |
Catamarca | 1.30 | lla | 1.85 | 1.8 |
Córdoba | 1.30 | llla | 1.85 | 2.0 |
Corrientes | 7.60 | lb | 1.85 | 1.8 |
Resistencia-chaco | 5.90 | la | 1.85 | 1.8 |
Paraná-entre ríos | 3.50 | llb | 1.85 | 1.8 |
Formosa | 7.70 | la | 1.85 | 1.8 |
Jujuy | 0.20 | llb | 1.85 | 1.8 |
La rioja | 0.40 | la | 1.85 | 1.8 |
Posadas-misiones | 6.90 | lb | 1.85 | 1.8 |
Santiago del estero | 2.10 | lla | 1.85 | 1.8 |
Tucumán | 2.20 | llb | 1.85 | 1.8 |
Oliveros-santa fé | 1.30 | llb | 1.85 | 1.8 |
Orán-salta | 4.9 | llb | 1.85 | 1.8 |
Cdro. Rivadavia-chubut | -1.10 | v | 1.74 | -- |
Santa rosa-la pampa | -2.70 | lla | 1.61 | 2.0 |
Mendoza | -1.00 | lva | 1.75 | 2.0 |
San juan | -1.50 | lll | 1.71 | 2.0 |
San luis | -0.70 | llla | 1.78 | 2.0 |
Neuquén | -7.3 | lvb | 1.33 | 2.0 |
Río callegos-santa cruz | -12.4 | vl | 1.11 | -- |
Barilocherío negro | -11.4 | vl | 1.15 | -- |
Ushuaia-tierra del fuego | -8.6 | vl | 1.23 | -- |
Source: IRAM 11.603, (2012).
Table 5 shows the maximum values of K according to the bioenvironmental zone defined in IRAM 11.603 and the level of thermal comfort. Buildings in bioenvironmental zone V and VI do not require cooling.
Table 5 Maximum thermal transmittance values according to the bioenvironmental zone and the level of hygrothermic comfort.
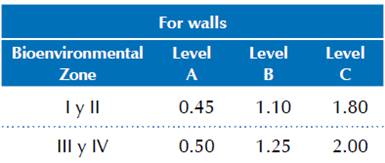
Source: IRAM 11605 (1996, p. 7).
Based on Level C comfort and the maximum admissible thermal transmittance K values for the winter season, Table 6 shows which materials are thermally suitable for use in the construction of enclosures within the various bioclimatic zones of Argentina as established by IRAM 11.603.
Table 6 Bioenvironmental zone certification of the thermal transmittance of various materials
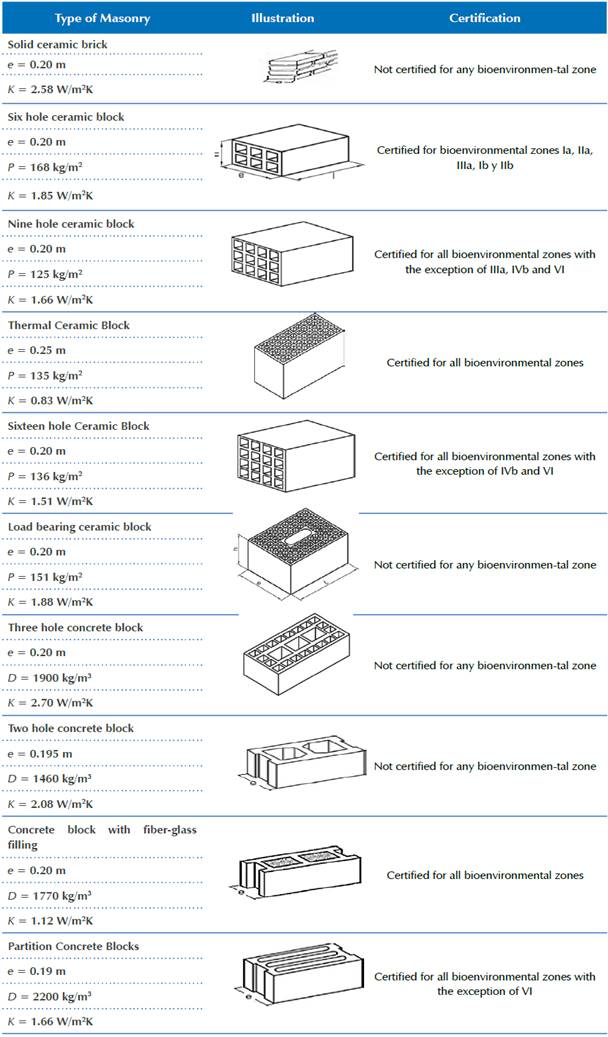
Source: author’s elaboration, based on IRAM 11.601 (2002, p. 14).
For this analysis, the most common examples of traditional construction were considered: 0.20 m thick brick wall, using 0.18 m wide bricks with 0.01 m of plaster on both sides, and concrete blocks were without plaster. In both cases, the surface resistance values were 0.13 m2K/W for the interior and 0.04 m2K/W for the exterior.
The plastered brick wall described above pro-vided a K value of 2.58 W/m2K, which does not meet the requirements for any bioenvironmental zone. If its thickness is increased to 0.30 m, the value of K decreases to 2.03 W/m2K, adequate only for summer conditions in bioenvironmental zones Illa, IVa and IVb, which are marked as very warm and warm areas (see Figure 4). In the case of a wall made of concrete blocks filled with fiberglass, it has a thickness of 0.19 m, which is certified for all bioenvironmental areas. This is the optimal option for winter.
Estimates for the Thickness of the Exterior Wall According to the Earthen Construction Technique Employed
Based on the analyses conducted by different authors and standards, five earthen construction techniques were chosen: adobe, CEB, rammed earth, wattle and daub and straw planking. An estimation was made for the minimum width of a load-bearing or free-standing exterior wall that would meet the certified K values for Level C comfort (see Table 7) for a house located in Greater Buenos Aires, bio-environmental zone lllb (a warm temperate zone with small thermal amplitudes throughout the entire year). In the case of adobe and CEB, exterior wall thickness varies from 0.35 m to 0.43 m. For rammed earth it is necessary to work with wall thicknesses of 0.40 m. As for wattle and daub and straw planking, both of which are more insulating due to the cane in wattle and daub and the air between the straw in straw planking, both allow for a reduced thickness. For wattle and daub, the external walls must 0.28 m thick. In the case of straw planking, the required wall thickness is 0.25 m. Furthermore, in the extreme case of the town of Río Gallegos, it was shown that the thickness of the wattle and daub wall must be 0.27 m to stay within Level C of the standard, yet a comfort Level B can be achieved with a total thickness of only 0.13 m by incorporating 2 cm of expanded polystyrene (Cuitiño, Esteves & Rotondaro, 2014). Observing these values, it can be concluded that, for Greater Buenos Aires, enclosures using one of these five techniques would be an acceptable thermal alternative to the ceramic brick enclosure with a thickness of 0.35 m.
Table 7 Estimates of the minimum necessary thickness of the outer wall to be certified for level c hygrothermal comfort in bioclimatic zone lllb (central area of Buenos Aires province).
Technique / type of wall | Thickness of exterior wall (m) | ||
---|---|---|---|
Load bearing | Free standing | ||
Adobe (density of 1500 y 1600 kg/m3) | Single | 0.40 | 0.40 |
Double | 0.43 | 0.43 | |
Ceb of 0,14 x 0,29 x 0,096 m (density of 1700 y 2000 kg/m3) | Single | 0.43 | 0.35 0.43 |
Double | 0.35 | 0.35 | |
Rammed earth (density of 1800 y 2000 kg/m3) | 0.40 | 0.40 | |
Wattle and daub (density of 700/900 kg/m3) | 0.28 | ||
Tamped-straw planking (density of 500/700 kg/m3) | 0.25 |
Source: author’s elaboration(2018).
Mechanical Strength of Materials and Elements of Enclosure Walls
There is a recurrent discrimination against earthen construction due to a lack of knowledge regarding the mechanical characteristics of its materials, components and construction systems. Many authors have carried out tests on adobe, CEB and rammed earth constructions to determine resistance to simple compression, cutting and bending stresses. This behavior is of the utmost importance when designing and constructing. Upon reaching the stage when the resistance of the constructive elements is to be evaluated, the construction system technique, the materials and the proportions become relevant.
Adobe Peruvian Standard E.080 (Ministry of Transportation, Communications, Housing and Construction, 2000) defines adobe as a "solid block of uncooked earth that may contain straw or other material to improve its stability against external agents and reduce cracks caused by shrinkage upon drying." In the case of CEB, the process is more controlled because a press is used to generate the compaction pressure, unlike in adobe masonry, which is not manufactured with mortar compaction. This compression implies an increase in the density of the block, which gives it superior mechanical qualities.
Rammed earth differs from the previous components because it is produced using a moveable formwork where the stabilized earth is compressed in layers, by means of a tamper, and in this way the wall is constructed in parts. Tables 8, 9, 10 and 11 present values obtained by various authors for compressive, flexural and tensile strength based on standardized tests in different countries and their own data.
Table 10 Mechanical resistance values for compression of rammed earth
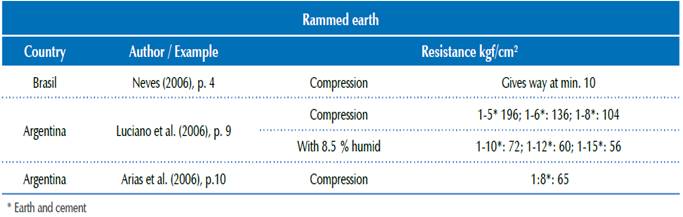
Source: author’s elaboration (2018)
Table 11 Shearing and simple compression resistance values for earthen and industrial construction materials and components (regalement cirsoc 501)
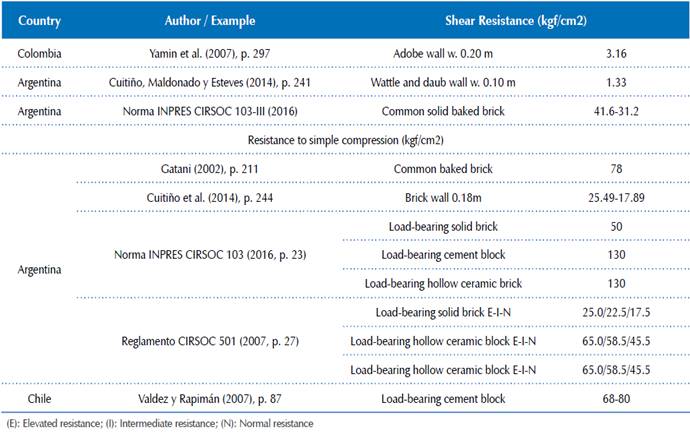
Source: autor's elaboration (2018).
In the case of adobe, the compressive strength varies between 3 kgf/cm2 and 21 kgf/cm2; its tensile and shear strengths are very low: 3.16 kgf/cm2. CEB shows an improved response with values ranging from 17 kg/cm2 to 121.8 kg/cm2. The oscillation reflects the cement content in the mixture: as the aggregate percentage of cement increases, the resistance to compression and bending increases. Finally, rammed earth has a variable resistance according to the sand-clay mixture and the thickness of the wall.
Thus, the values obtained vary between 46 kgf/cm2 and 196 kgf/cm2. In the case of other materials and components, such as common fired brick, Table 12 shows compression values from 17.5 kgf/cm2 to 78 kgf/cm2. For hollow concrete block, these values range between 45.5 kgf/cm2 and 130 kgf/ cm2. According to these data, it can be seen that adobe has very low values of mechanical resistance, so it would be necessary to reinforce the construction to improve its structural behavior. CEB and rammed earth have a better response than adobe in terms of standardized mechanical strength values, and they can be framed between the responses of common fired brick and concrete blocks. However, despite its best response, it should be borne in mind that, in the case of manufactured systems, it is about 0.18 m thick, and in the case of earthen construction systems, it is about 0.30 m and 0.90 m thick.
Discussion
This study provides a comparative analysis of the thermal and mechanical behaviors of various construction materials and elements made from stabilized natural soils in relation to the properties of some conventional industrialized materials. It shows the difficulty in homogenizing the values for adobe, light-weight soil and rammed earth techniques. This behavior is a result of the variable densities and the range of materials and mortars typically used in their manufacture.
It was also shown that the thermal conductivity varies exponentially with respect to density, which changes according to the presence of vegetable fiber and the degree of compaction. That is, the greater the compaction, the lower the porosity, or percentage of air pockets; thus, the insulation also decreases and thermal conductivity increases: the higher the density of the construction element, the greater the conductivity value. This behavior is probably the origin of the differences, sometimes notable, between thermal conductivity values, or thermal transmittance values, in the results obtained through the standardized tests that different authors conducted. Using data from Tables 1 and 2, Table 13 summarizes the thermal behavior by providing the range of values for density, conductivity and thermal transmittance, regardless of the authors who conducted the original studies.
It can be seen that the density values for materials used in earthen construction techniques that possess low fiber density mortars or compacted stabilized soils have values between 1200 kg/m3 and 2200 kg/m3. It is not possible to work with lower densities because doing so implies the existence of more air incorporated into the mortars.
In the case of the most common industrialized materials in conventional Argentinian construction, it can be seen that they possess higher densities: between 1305 kg/m3 and 2400 kg/m3.
Regarding IRAM Standard 11.605, it can be concluded that 1.85 W/m2 K are necessary to achieve environmental comfort Level C in zone lllb, the Province of Buenos Aires. Thus, to achieve that level of insulation with conventional technology, walls made with common solid brick require a thickness of 0.35 m, and in the case of hollow ceramic blocks and load bearing ceramic blocks, a wall 0.20 m thick is needed. Comparatively, the necessary thicknesses estimated for the exterior walls of adobe and CEB range between 0.35 m and 0.43 m; for rammed earth, a wall of 0.40 m is needed; in the case of wattle and daub, a thickness of 0.28 m is needed; and for straw planking, a thickness of 0.25 m is needed.
The other behavior under analysis was mechanical resistance, primarily to simple compression, because it is one of the most important mechanical properties of earthen materials and building components. In the case of simple compressive strength, the range or variation is between 3 kgf/cm2 and 21 kgf/cm2 for adobe, except for a single value of 30.4 kgf/cm2 in Table 9, which shows very low tensile and shear resistance. The simple compressive strength improves in the case of the CEB, with values between 17 kg/cm2 and 121.8 kg/cm2, comparable to those of common fired brick, which can range from 17.5 kg/cm2 to 70 kg/cm2, and those of hollow concrete blocks, which are between 45.5 kgf/cm2 and 130 kgf/cm2.
Conclusions
As a final reflection, and considering the results of this research, even taking into account the distinctive features and limited behavioral standardization for different materials, mortars and construction elements, the projected development of earthen construction and architecture in Argentina is very relevant in the field of housing. This projection is based on the fact that, in recent decades, there has been an increase in the use of this technology to produce homes and public buildings throughout Argentina, from regions with the highest seismic vulnerability to those with the lowest. In the near future, these changes could contribute to an improvement in offers for the construction of habitats, with a clear orientation towards sustainable architecture, as well as a reduction in the housing deficit.
It is possible that this increase in constructions with earthen technology has been influenced by the inherent characteristics and properties of their mechanical and thermal behaviors, such as insulating capacity, ease of construction, the use of natural local materials, and a low relative economic expense.
Likewise, the structural aspect of some earthen construction techniques can be beneficial if they are met with quality design and execution regarding the wall width, reinforcements and proportionality. This is relevant for seismic zones, since this technology offers the possibility of producing earthen constructions with light, flexible and resistant structures.
The greater acceptance of and interest in earthen construction are an incentive to continue investigating the advantages of its thermal properties and characteristics and the mechanical resistance that characterize the different construction methods that use modified natural soils and buildings of increased environmental comfort and structural stability