1. Introduction
Undoubtedly, by the mere fact of existing, one can infer that the interaction between the geological evolution of planet Earth (including the climate) and the evolution of life has been favorable. However, this assertion stands because planet Earth is the only place we know that harbors life as it is, in the entire universe. Nevertheless, a recent update of the very famous Drake equation (Drake, 1961) supports the fact that the probability of finding life on another planet should not be zero (Gertz, 2021).
Climate forcing factors can be divided into terrestrial and extraterrestrial (Frakes et al., 1992). Terrestrial forcing includes various mechanisms such as atmospheric greenhouse gases, the geochemistry and biochemistry of the oceans, land-sea distributions and their effects on global albedo, and geographic restraints through changes in topography, ocean circulation and the formation of bodies of water. Extraterrestrial forcing deals with changes in solar luminosity, passaging the solar system through matter clouds in space, bolide impacts, and changes in the Earth's rotational speed and orbital parameters. States with a high concentration of greenhouse gases generally correspond to high levels of atmospheric CO2 and increased warming. These phases are linked to changes in sea level and volcanism. In most volcanic episodes, there seems to be a relationship with these hot or cold modes (Hartmann, 2016). Therefore, volcanic activity could be related to the termination of cold modes (e.g., early Permian, early Cretaceous), reinforcing warming trends in other periods (e.g., early Ordovician, Devonian, and late Cretaceous). Although the contribution of greenhouse gases (mainly CO2) by volcanic activity during geological time is uncertain, there is a hypothesis that the global climate is influenced and perhaps forced by volcanic exhalations (Hartmann, 2016; Racki, 2020). Another important event associated with cold and warm modes is carbon peaks in the ocean, which occur in cold or glacial conditions, and are reported to be associated with marine extinction (Wilde & Berry, 1984). As we will see later, most of the time biotic crises occurred during warm periods.
In this way, the evolution of the Earth's atmosphere and climate has not always been entirely "beneficial" for the development of the multiple species that have inhabited this planet. It is convenient to use the geological time scale as an appropriate frame of reference to describe the climate changes and their impacts on biodiversity arranged chronologically (Cohen et al., 2013). Figure 1 shows a synthesis of the international chronostratigraphic chart, the updated version of which can be obtained online.
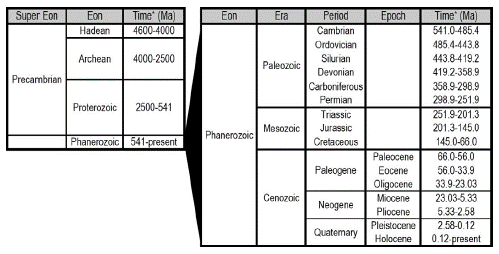
Figure 1 Geological time summarized from the official international chronostratigraphic chart1. * Time is the duration of the category in question in millions of years (Ma) regarding the present.
This article presents an overview of some of the major discussions related to the evolution of the climate (throughout the different geological eras) and its potential impact on mass extinction events. In addition, we include a discussion (Section 5) of how estimated future climate changes (according to the Intergovernmental Panel on Climate Change, IPCC) compare to past climate changes. As noted by (Frakes et al., 1992) (p. 1) "... only by understanding past climate states of the Earth can we discern the driving mechanisms for global climate change, set boundary conditions for numerical modeling and learn how to predict future climates".
2. The Precambrian Super Eon
The solar system was created about 4,600 Ma after the collapse of a molecular cloud that gave rise to the solar protostar and the subsequent formation of planets (Prentice, 1979; Alfvén, 1982). According to the Great Impact hypothesis, about 4,500 Ma the original planet Earth collided with another smaller planet, known as Tea, giving rise to the Earth and Moon that we know today (Canup, 2012).
After its formation, the Earth must still have stored a great deal of heat, giving rise to ubiquitous volcanism. The gases emitted by the volcanoes gave rise to the formation of the primitive atmosphere, whose components could be carbon dioxide and water vapor (from volcanoes) and molecular hydrogen (from the solar nebula), which must have been very unstable because of the loss of light gases into space (Pla-García & Menor-Salván, 2017).
During this time, other physical processes also occurred that were later fundamental in the development of life. Some suggest that the Earth's crust formed as early as 4,400 Ma and, although no rock evidence is found in this period, some remains of the mineral zircon have been dated to that time (Valley et al., 2014). It is likely that the oceans formed during this eon, although little is known about their possible extent (Morse & Mackenzie, 1998). However, some theories mention the possibility of a wider oceanic coverage than the current one, as a factor that prevented the Earth from freezing during its early stages of development when the solar intensity was 30% lower than the current one according to some theories of stellar evolution (Kasting, 1993; Wicander & Monroe, 2016). The other important factor refers to the existence of a greater amount of greenhouse gases that allowed the global average temperature to be more suitable for the development of life. Later came the stage of the Late Heavy Bombardment (between 3,800 and 4,100 Ma) in which many meteorites fell on Earth (and the other inner planets) (Cohen et al., 2000; Claeys & Morbidelli, 2015; Bottke & Norman, 2017). The end of the Late Heavy Bombardment coincides with the first isotopic tests of life (Mojzsis & Harrison, 2000), although the uncertainty remains whether life really arose at that time or had already arisen before and survived the bombardment (Maher & Stevenson, 1988; Chyba, 1993).
The Archean Eon covers almost a third of the whole geological time (Figure 1) and is the one during which there is irrefutable evidence of the existence of the first rocks on the planet. In fact, the oldest rocks, almost 4,000 Ma, are found at Acasta Gneiss in the Northwestern Territories of Canada (Wicander & Monroe, 2016).
Likely volcanoes during the Archean Eon emitted gases in the same way as they do today, so the atmosphere must have contained mainly water vapor, sulfuric dioxide, carbon dioxide and monoxide, whose reactions would produce ammonia and methane; however, molecular oxygen and ozone were lacking (Kasting, 1993). These conditions persisted during the Archean (Wicander & Monroe, 2016). There are two processes by which free oxygen is thought to have entered the early atmosphere. The first is photochemical dissociation, in which ultraviolet sunlight breaks water molecules high in the atmosphere, releasing hydrogen and oxygen. Around 2% of today's oxygen may have been formed by this process; however, it was enough to cause the formation of the stratospheric ozone layer. The second important process is photosynthesis, which could contribute to the formation of atmospheric oxygen until there were organisms with this metabolic process in which they used carbon dioxide and water to produce organic molecules and released oxygen as a waste product. Multiple lines of geochemical evidence show that O2 was produced by cyanobacteria on the ocean surface, and these metabolizing organisms were present in abundance in the latter part of the Archean, and most likely as early as 3,500 Ma (Kendall et al., 2010; Wicander & Monroe, 2016; Ostrander et al., 2019).
Most of the known Proterozoic organisms are unicellular prokaryotes (bacteria). There is still uncertainty about when eukaryotic cells first appeared, but it is likely that they were present for at least 1.2 Ma (Wicander & Monroe, 2016). The oldest known multicellular organisms are probably algae, some of which may date back to the Paleoproterozoic (~ 1,800 Ma).
Since the beginning of the Proterozoic Eon, 2,500 Ma, the Earth's climate has fluctuated between cold (Earth covered mostly by ice) to greenhouse (Earth free of ice) conditions. Since then, there have been at least five great Ice Ages lasting between 30 and 215 Ma. In the other 70% of the time, the Earth has experienced ice-free greenhouse conditions, even at the poles (Crowley, 1995).
At the beginning of the Proterozoic Eon, perhaps the first great mass extinction event occurred (although it is not counted within the "famous five" which all occurred in the Phanerozoic Eon, see Figure 2); this event is known as the Great Oxidation, which occurred about 2,400 Ma (Margulis & Sagan, 1997; Gumsley et al., 2017; Ostrander et al., 2019). At first, the excess oxygen was absorbed in chemical reactions with iron or by decomposing cells, however, the population of cyanobacteria increased due to their same ability to provide energy by increasing the amount of oxygen that was greater than what could be absorbed, annihilating most anaerobic organisms, for which oxygen is toxic (Schirrmeister et al., 2013; Schirrmeister et al., 2015; Ostrander et al., 2019; Sánchez-Baracaldo et al., 2022).
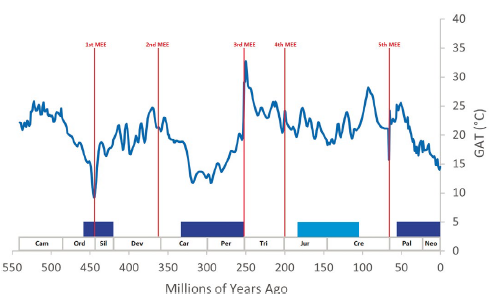
Figure 2 Phanerozoic global average temperatures (GAT) as reported by Scotese et al. (2021). Blue and turquoise rectangles show the periods of three great Ice Ages (in blue) and one cool period (in turquoise) according to Frakes et al. (1992). Finally, the five mass extinction events (MEE) are shown as red lines according to Bond & Grasby (2020).
In addition, with the chemical reactions of oxygen and methane, which oxidized with ultraviolet radiation and produced carbon dioxide, the global temperature would be considerably reduced because of the greenhouse effect. This impressive climate change would trigger the Huronian Glaciation (2,290 Ma to 2,250 Ma), one of the most intense that has existed and from which few organisms escaped, even almost extinguishing the cyanobacteria themselves. Most of the evidence for the appearance of this glaciation event may be related to the rupture of the Kenorland supercontinent, allied with a significant atmospheric change, as well as the emergence of biogeochemical oxygen photosynthesis (Tang & Chen, 2013). This turns out to be the first coincidence in which an extreme paleoclimatic event (in this case glaciation) is directly/ indirectly related to the loss of organisms on Earth (in this case anaerobic organisms and cyanobacteria) affecting biodiversity.
Towards the end of the Proterozoic, in the Cryogenic Period (~720 to ~635 Ma), there were multiple glaciations that have generated a strong discrepancy in the hypotheses about the causes and times in which they occurred (Kendall et al., 2006; Arnaud et al., 2011). For example, within the causes of these glaciations, especially the larger one known as Sturtian, is the hypothesis of the "Snowball Earth" in which the planet's surface was completely covered by a layer of snow (Hoffman et al., 1998; Hoffman & Schrag, 2002); another is the "Slushball Earth", where only a small equatorial strip remains free of snow on the surface (Hyde et al., 2000); and finally a generalized but regional and diachronic glaciation, during the prolonged rupture of the supercontinent Rodinia (Eyles & Januszczak, 2004; Kendall et al., 2006). The hypothesis of the quasi-frozen Earth except in the tropics (slush-ball) is very interesting because it proposes a more viable survival alternative for this critical period in the evolution of multicellular life than the "Snowball Earth" (Hyde et al., 2000).
Unlike living organisms, warm and cold modes appear to be independent of the boundaries of major geologic time intervals. However, the effect that global climate change has had on both the evolution and dispersal of organisms is undeniable and clear examples can be observed at shorter time scales (Frakes et al., 1992).
3. Extreme paleoclimate of the Phanerozoic Eon
The climate on Earth has had important variations throughout its evolution during the current Phanerozoic Eon (~ 541 Ma to the present), which precedes the entire Precambrian Super Eon (Figure 1). Its name is derived from the Greek and means "visible life". That is the period where multicellular organisms strengthened enough and grew to be remarkable on a human scale. It is also the time when the famous "five mass extinctions" and three of the five most important glacial periods have occurred (Figure 2). This section briefly discusses the potential connection between changes in hot and cold periods of the climate with the development or extinction of ecosystems. A recent review of this connection is shown by Bond & Grasby (2017) and summarized in Table 1 of that work.
The cycle of hot and cold periods during the Phanerozoic Eon is well established among the scientific community (Fischer, 1981, 1982, 1984; Frakes et al., 1992, fig. 11.1; Scotese et al., 1999; Summerhayes, 2015; Scotese et al., 2021) and is consistent with that stated in Figure 2. Multiple tectonic and environmental processes generally control long-term (>50 Ma) global temperature that drive Earth's climate from icehouse to hothouse conditions and back (Scotese et al., 2021). The dominant factors are the level of greenhouse gases (mainly CO2) (Grossman & Joachimski, 2022), which are regulated by the release of volcanic gases and CO2 extraction due to weathering (van der Meer et al., 2014), the geographic configuration of the continents and ocean basins (see Figure 3), and the Earth's albedo. An icehouse world is simply defined as a time when the Earth is covered by permanent ice at either pole. On the other hand, "hothouse" times arise when there is no ice above the polar circle - even during the winter (Scotese et al., 2021).
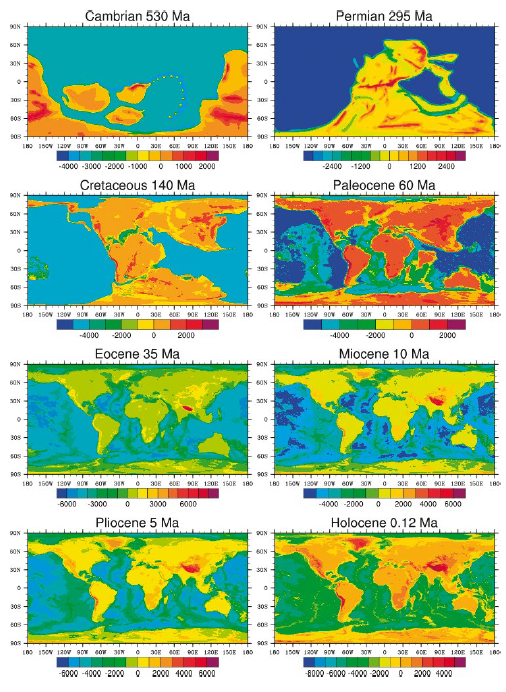
Figure 3 Evolution of the paleotopography and paleobathymetry (z, in meters above sea level) for different geological periods (Cambrian, Permian, and Cretaceous), and epochs (Paleocene, Eocene, Miocene, Pliocene, and Holocene) of the Phanerozoic Eon (data from Scotese & Wright, 2018).
In the early Cambrian, the late glacial phase of the Proterozoic ended, and the Earth warmed. The first discernible evidence of this is in the latitudinal expansion of carbonate sedimentation, but since the expansion was gradual, it is inferred that the process was slow and did not reach completion until the Middle Ordovician. The warm mode ended in the Middle Ordovician with the development of ice sheets in North Africa (Frakes et al., 1992).
The Ordovician Period had great oceanic diversification (Sepkoski, 1981), cut off by the first of the five great extinctions: the Late Ordovician mass extinction. Two pulses eliminated 85% of marine species (Jablonski, 1991) during this ecological crisis, the second most severe in the entire Phanerozoic (Bambach et al., 2004). The most accepted extinction scenarios of this crisis call for changes in ocean temperature and redox chemistry: the first pulse, at the beginning of the Hirnantian Age (-445 Ma ago), affected nekton and plankton and has been linked to the cooling at the beginning of the Gondwana glaciation (Brenchley et al., 2001); a million years later, the second pulse (still in the Hirnantian), is associated with warming, rising sea levels and anoxia (Nielsen, 2004). However, recently Bond & Grasby (2020) have proposed a novel idea, which is that the first pulse was also caused by volcanism, global warming and anoxia, and not by the third ice age associated with this period (Figure 2). In this way, instead of being the strange event among the five mass extinctions, this event is like the others in being caused by volcanism, heating, and anoxia; despite being immersed in a low frequency cold mode (Bond & Grasby, 2020). Figure 2 illustrates how the other four mass extinction events occurred under warm phase climate modes (Bond & Grasby 2017; 2020), as we will discuss below.
The second major mass extinction, which occurred in the Upper Devonian and early Carboniferous, between the Famennian (~ 370 Ma) and the Tournaisian (~ 355 Ma) Ages, was associated with global warming (+9 °C above the current average) imposed on cold pulses, caused by large volcanic events, a meteor impact (Siljan Ring), possible marine anoxia and changes in atmospheric carbon concentration (Bond & Grasby, 2017). Changes in ocean chemistry and a climate that cooled were the causes of this extinction in its last stage. This process occurred with the enrichment of 13C in the ocean, which may have been due to the photosynthesis-respiration process that removes 12C by organisms from the surface water and was deposited on the ocean floor, where there was enough oxygen by which combined and produced CO2. However, it was produced in large quantities that when oxygen was depleted, organic matter was removed (Frakes et al., 1992).
The third great mass extinction, at the end of the Permian (~ 252 Ma), is considered the most powerful of all, where up to 96% of existing species were lost (Sepkoski, 1984; Erwin et al., 2002; Li et al., 2021). It is also associated with even stronger global warming (+10 °C above the current average) caused by large-scale volcanism (the Siberian traps), marine anoxia, a decrease in the concentration of atmospheric carbon, probable ocean acidification and possible impacts by meteors. In addition, other factors such as acid rain, poisoning by toxic metals (Hg) and damage by UV-B radiation are associated (Bond & Grasby, 2017).
By the end of the Triassic (~ 200 Ma), the fourth major mass extinction event occurred, which is estimated to have been caused by global warming of +6 °C above the current global mean temperature due to large-scale volcanic activity, probable ocean acidification, decrease in atmospheric carbon levels and not related with a major meteor impact event (Bond & Grasby, 2017).
The peak of C in the Aptian (from 125 to 113 Ma) occurs near the end of the middle Jurassic to the cold mode of the Cretaceous. As the productivity of nutrients decreases, the CO2 increases, which contributed significantly to the warm period that characterizes the late Cretaceous. During the Aptian-Albian (from 125 to 100 Ma) the sea level increased due to global warming. The carbon spike in warm modes may not always be associated with high nutrient productivity caused by colder climates and upwelling, but may be associated with reinforced confinement under strongly anoxic conditions (Frakes et al., 1992).
Finally, the famous great Chicxulub impact, at the end of the Cretaceous (- 66 Ma), was the trigger for the fifth and last great mass extinction that caused the decline of dinosaurs and many other species (76% of species perished). Besides this, the event occurred in a period of a warm mode with global warming of +4 °C caused by strong volcanic activity (the Deccan traps), a slight decline in the concentration of atmospheric carbon, and poisoning by toxic metals (Hg) (Bond & Grasby, 2017). This is the last great mass extinction event, although some others speculate that the sixth great extinction has already started since the Anthropocene (Pievani, 2014).
Cowie et al. (2022) provide an updated discussion of the last argument above. They point out that currently the scientific community splits in those who are in favor that the sixth mass extinction is already underway (this time caused entirely by humans), and those who, even though considerable evidence supports a biodiversity crisis of increasing extinctions and decreasing abundances, do not accept that this amounts to a sixth major extinction. One of the key points on the discussion arises because the famous International Union for Conservation of Nature's Red List of Threatened Species (IUCN) Red List2 shows that the rate of species loss does not differ from the background rate. However, the list is heavily biased because almost all birds and mammals, but only a minute fraction of invertebrates, have been assessed against conservation criteria. Incorporating estimates of the actual number of invertebrate extinctions leads to the conclusion that the rate far exceeds the background rate (Cowie et al., 2022).
4. Extreme paleoclimate of the Cenozoic Era
The onset of the conditions that culminated in the Quaternary glaciation (Pleistocene and Holocene series) resulted from the long-term cooling trend in world climates that began from the beginning of the Cenozoic Era, during the Paleogene Period (see Figure 2), where the last ice age that has survived to this day began (Ehlers et al., 2011). Besides some limited activity in the Eocene, in both the southern and northern hemispheres (Stickley et al., 2009), significant glaciation began in the latest Eocene-early Oligocene (~ 35 Ma) in eastern Antarctica (Ingólfsson, 2004). It was followed by mountain glaciation through the Miocene (23 to 5.3 Ma) in Alaska, Greenland, Iceland, and Patagonia, and later in the Pliocene (5.3 to 2.6 Ma) in the Alps, the Bolivian Andes, and possibly in Tasmania. From Neogene, glacial-derived raft ice remains are found in oceanic sediment cores in the North Atlantic region, including the Barents Sea (Moran et al., 2006) and areas next to Norway, northern and southeastern Greenland, Iceland and northern North America, and in the Southern Ocean off Antarctica (Ehlers et al., 2011).
A link between climate change and human evolution during the Pleistocene is often assumed but has rarely been proven (Gamble et al., 2004; de Menocal, 2004; Maslin & Christensen, 2007; Maslin & Trauth, 2009).
Homo appears on Earth approximately between 2.5 and 3 Ma. During this time range in Africa, many areas became grasslands as the climate became drier. This could be the reason humans went from sedentary to nomadic. The state of the climate, in that and other times, is derived from sediment studies. The dry periods are determined by the dark, dirty, and sandy layers that expel the sea by the manifestation of monsoons, while in the wet periods, the layers contain light amalgamations of abundant fossilized plankton. These characteristics dimension the conditions in which the terrestrial environment was in the temporalities described above. In this period, there were also rapidly variable climate oscillations (on a geological time scale). Records of this climate were found in Africa, which is known as the paleoclimatic record of the Valley. Recently, Rousseau et al. (2022) found that orbital and millennial-scale variability appear to interact during the Quaternary, with millennial variability increasing in intensity as the ice sheets grew in both area and volume. Thus, sudden changes in climate are undoubtedly related, albeit indirectly, to the astronomical theory of climate (Milankovitch theory). Homo then witnessed unfamiliar landscapes during these changing times, where diverse food options were presented, while the environment tended towards a more uniform grassland landscape. During humid periods, such as the Middle Stone Age (about 40,000 to 80,000 years ago), the climate transformed to humid conditions in South Africa. Homo gave rise to the innovation of language and its own cultural identity (Handwerk, 2014). According to dynamic simulations, the severe cooling and expansion of the ice sheets during the Last Glacial Maximum, 27,000-19,000 years ago had a great impact on the populations of plants and animals, including humans (Tallavaara et al., 2015).
Agriculture emerged 10,000 years ago and coincides with the end of the last ice age since we are currently in an interglacial phase. The earliest human brains, which were smaller but significantly different, are believed to have occurred when periods of climate were more stable. So, climate changes have influenced the adaptation of humans, animals, and plants. Climate has also been a factor that may have influenced human migrations from Africa to Europe and Asia. The Neanderthal and Denisovan may have originated from former groups like Homo Heidelbergensis, which became isolated and lived in the Northern Hemisphere. The process at the equator is believed to differ from this, since perhaps the climate in that region was not so extreme (Handwerk, 2014).
Next, we discuss how the climate changes of the past compare to the latest projected scenarios reported by the IPCC.
5. Updated historical and future climate change scenarios as reported by the IPCC
The previous sections have briefly reviewed some effects of the past climate on biodiversity, where terrestrial and extraterrestrial climate drivers have been pointed out that have determined the cold and warm states to which the Earth is subjected and the processes in which the chemical composition of the atmosphere and ocean have been changed to extreme conditions. Regarding an atmosphere with high concentrations of CO2, the estimates during the Quaternary Period (Pleistocene and Holocene, ~ 2.5 Ma), give values between 300 and 500 ppm. The sea level was 20 m higher, and the temperature was approximately 2 °C higher than today (Frakes et al., 1992). CO2eq concentrations were between 300 ppm and 700 ppm in the late Miocene (~ 10 Ma). For the rest of the Cenozoic (Paleogene > 30 Ma) these concentrations were between 700 ppm and 1000 ppm. Previously during the Mesozoic, CO2 concentrations were reduced to less than 500 ppm (IPCC, 2013). It is possible that the human being will face for the first time a high level of CO2 concentrations that is expected for the following decades. However, for the rest of the ecosystems, these types of levels are not at all alarming and they will surely survive as they have been doing for the last 500 million years (Frakes et al., 1992).
This serves as the basis for trying to assess the potential effects of the climate in the decades to come. Earth's system models (ESMs) are currently the most complete tools to simulate the Earth's climate at different periods. ESMs simulations are primarily determined by the internal and external forcings on the models. Factors such as the flux of solar radiation that our planet receives are among the most prominent, but other factors, such as the distribution of the continents, the topography of the Earth, the bathymetry of ocean basins, the availability of chemical elements and many others, they also play an important role (Feulner, 2009). ESMs are used to determine the changes in these factors in the climate and to generate the IPCC climate change scenarios diversified under the characteristics of CO concentrations and the radiative forcing levels at 2100 (IPCC, 2013).
The IPCC-AR5 set of scenarios, better known as Representative Concentration Pathways (RCP), characterize the development and modeling of the physical components of the climate worldwide in the Coupled Models Intercomparison Project Phase 5 (CMIP5) (Moss et al., 2008). These scenarios are classified into RCP2.6 (optimistic), RCP4.5 (conservative CO2eq mitigation), RCP6.0 (significant increase in CO2eq concentrations and moderate mitigation), RCP8.5 (does not perform any mitigation activities CO2eq). In addition, all of them coincide with an increase in surface temperature that will exceed 1 °C. With exceeding 1.5 °C, there is still a consensus with a high probability for all scenarios, except RCP2.6, which reports a medium probability. To exceed 2 °C there is a high probability that it will happen only under the RCP6.0 and RCP8.5 scenarios, while it is moderately probable with RCP4.5 and less probable with RCP2.6. All scenarios report an increase in temperature due to the amount of CO2 concentrations that are already in the atmosphere. This increase will occur in 2050 for some scenarios or by 2100 with others, where CO2eq concentrations in the atmosphere would be between 500 ppm and 1000 ppm. These RCP projections allow us to know the possible climatic conditions of the Earth for the coming years for different CO2 emission scenarios and in this way better prepare us to face the potential impacts of climate change. Information from the IPCC-AR5 (IPCC, 2013) shows that the expected global warming of the most drastic scenario (RCP8.5) is far from reaching the maximum global warming of the planet throughout its history (~10 °C above present value); however, the real problem is that this warming is expected to occur in a geologically very short period. Past changes in the Earth's temperature and CO2 concentrations took place over hundreds (if not thousands) of years; while the changes we are currently observing take place over one hundred years (i.e., during the present century). This brings with it the fact that ecosystems and humanity have very little time to adapt to these new temperatures and the consequent changes in climate extremes that this entails.
After year 2100, CO2eq concentrations are expected to exceed 500 ppm including above 1500 ppm for the most drastic RCP8.5 scenario, while surface temperature increases exceed 3 or 4 °C (IPCC, 2013). Similar CO2eq concentrations have been present in the Earth at behind years, as described in section above. Hansen et al. (2013) report that the climate sensitivity is 3 ± 1 °C for 4 W/m2, 2x CO2 forcing, i.e. (0.75 °C per W/m2). However, the CO2 fraction is 55% since 1950, this means 5000 GtC are required to yield 9 W/m2, close to RCP8.5, that magnitude would make most of the planet uninhabitable by humans.
The new Phase 6 Coupled Models Intercomparison (CMIP6) project (Eyring et al., 2016), has already mixed RCPs with new socioeconomic scenarios, known as Shared Socioeconomic Pathways (SSP), in such a way that there are comprehensive scenarios for the development of both mitigation and adaptation actions appropriate to climate change. In this way, the issue continues to be of primary importance to the agenda of the scientific community and decision makers.
The new IPCC Sixth Assessment Report (IPCC-AR6) shows that greenhouse gas emissions vary depending on the socio-economic scenario. The scenarios with the highest probability of temperature increase (> 1.5 °C for the last decades of the present century) are SSP2-4.5, SSP3-7.0 and SSP5-8.5 (IPCC, 2021). The IPCC-AR6 reports that the increase in global warming leads to changes in major extreme hydrometeorological events. For every 0.5 °C more warming, the frequency of extremes of heat, such as warmer days and heat waves, as well as higher rainfall, increases. More intense agricultural and ecosystem droughts also occur in some regions (IPCC, 2021). These changes in the climate due to global warming are shown in short periods and are reflected in hydrometeorological phenomena, implying that reaction actions to these events must be dynamic and possibly out of reach to address the impacts they cause in social and ecological systems.
The likely range of total anthropogenic increase in global surface temperature between 1850-1900 and 2010-2019 is 0.8 °C to 1.3 °C, with an estimated mean of 1.07 °C (IPCC, 2021); that rate is unprecedented in at least the last 2000 years. The estimated temperature (very likely range) during the warmest multi-century period in at least the last 100,000 years, is 0.2 °C to 1.0 °C, which happened around 6500 years ago during the current interglacial period (Holocene) (IPCC, 2021; Gulev et al., 2021) (see Table 1). In 2019, atmospheric CO2 concentrations reached a level higher than in at least 2 million years (high confidence), and concentrations of CH4 and N2O were higher than in at least 800,000 years (very high confidence) (IPCC, 2021).
Table 1 Global Temperature evolution over the past 50 Ma, present and future projections. Source: Gulev et al. (2021).
AGE | TIME | GLOBAL TEMPERATURE ANOMALY RELATIVE TO 1850-1900 (°C) | DATA* |
---|---|---|---|
PALEOCEN-EOCENE | 50,000,000 Before | ~ 15.0 | Proxy |
PALEOCEN-EOCENE | 35,000,000 Before | ~ 3.0 | Proxy |
MIOCENE | 15,000,000 Before | ~ 7.0 | Proxy |
MID-PLIOCENE | 1,000,000 Before | ~ 0.0 | Proxy |
PLEISTOCENE | 500,000 Before | ~ -1.0 | Proxy |
PLEISTOCENE | 100,000 Before | ~ 2.0 | Proxy |
PLEISTOCENE | 20,000 Before | ~ -3.0 | Proxy |
HOLOCENE | 2000 Before | ~ 0.5 | Proxy |
PRESENT | 0 | ~ 1.0 | Instruments Records |
FUTURE PROJECTION | 2100 | ~ 5.0 | SSP5-85 Scenario** |
FUTURE PROJECTION | 2300 | ~ 14 | SSP5-85 Scenario |
*See Gulev et al. 2021 for detail.
**CMIP6 scenarios.
The latest projections give a best estimate for the increase of the global mean temperature of 1.4 °C (very likely range 1.0 to 1.8 °C) for the most conservative case (SSP1-1.9) during the long-term period (2081-2100), up to 4.4 °C (very likely range 3.3 to 5.7 °C) for the worst-case scenario (SSP5-8.5) in the same period (IPCC, 2021). These projections show extreme events are likely to intensify in the coming decades, such as the global water cycle and its variability, global monsoon precipitation, and the severity of wet and dry events. Many changes due to past and future greenhouse gas emissions are irreversible over centuries or millennia, especially changes in the ocean, ice sheets, and global sea level (IPCC, 2021; Chen et al. 2021) (see Table 2).
Table 2 Paleoclimatic reconstructions of atmospheric CO2 concentrations. Global mean sea level over years past to present to CMIP6 projections. Source: Chen et al. 2021 (Figure 1.5).
TIME RELATIVE TO 1850 | ATMOSPHERIC CO2 CONCENTRATION | GLOBAL MEAN SEA LEVEL |
120 000 YEARS BEFORE | ~250 ppm | ~ -90 m |
1,000 YEARS BEFORE | ~200 ppm | ~-120 m |
0 | ~290 ppm | ~0 m |
170 YEARS AFTER (2020)* | ~410 ppm | ~0.1 m |
250 YEARS AFTER (2100)** | ~1600 ppm | ~1 m |
450 YEAR AFTER (2300)** | ~2500 ppm | > ~5 m |
*2020 year.
**Future projections from CMIP6 data under the SSP5-85 scenario.
CMIP6-Endorsed Model Intercomparison Projects (MIPs) have a broad coverage and distribution across the CMIP6 science question linked to the World Climate Research Program (WCRP). One major project which certainly helps to assess the performance of climate models to simulate past climate changes and compare them with those in the future is the Paleoclimatic Modeling Intercomparison Project (PMIP). Its main objective is to (a) analyze the response to forcings and main feedbacks for past climates outside the range of recent variability; and b) assess the credibility of climate models used for future climate projections (Eyring et al., 2016, Gulev et al., 2021). PMIP also addresses how well state-of-the-art numerical models simulate climate change, through comparison with observations of the environmental impact of these climate changes, or with climate reconstructions based on physical, chemical, or biological records (WCRP, 2022).
Five different periods have been designed in the latest phase of PMIP (PMIP4) to contribute to the objectives of CMIP6: the millennium prior to the industrial epoch (past1000), the mid-Holocene, 6,000 years ago (midHolocene); the Last Glacial Maximum, 21,000 years ago (lgm); the Last Interglacial, 127,000 years ago (lig127k) and mPWP, the mid-Pliocene Warm Period, 3.2 million years ago (midPliocene-eoi400) (WCRP, 2022)3. Kageyama et al. (2021) compare the latest paleoclimate climate model simulations (CMIP6-PMIP4) to the previous one (CMIP5-PMIP4) and to reconstructions. They show that large-scale climate features (e.g. land-sea contrast, polar amplification) are well captured by all models, while regional changes (e.g. winter extratropical cooling, precipitations) are still poorly represented.
6. Final comments
One of the key questions to resolve is whether the climate change generated by humans in recent decades is something that will last for a long time or if it will rather be a short-term increase seen from a geological perspective (Wicander & Monroe, 2016). Recent geological evidence supports the fact that, although humans are accelerating global warming, the fact that we are in an interglacial period will cause the planet, within a few thousand years, to return to an ice age. However, global warming will continue to affect ecosystems in the coming decades, something that has been observed both in the past and recently (Gamble et al., 2004; Moss et al., 2008).
These higher warming scenarios, especially in such a short geological period, have put virtually everyone on alert. For almost three decades now, climate change has been on the international policy discussion agenda to implement mitigation measures to reduce greenhouse gas emissions into the atmosphere and implement adaptation measures that achieve minimize the serious consequences that are expected from global warming in the decades to come. After all, as we have seen here, the great mass extinctions on the planet have been directly related to global warming events, either long or relatively short term (Bond & Grasby, 2020). This result should be one more alert that humans must implement drastic measures, such as those implemented in the current COVID-19 pandemic, to make actual life changes such that they make us more efficient in using energy and electricity and implement technologies that are less aggressive to the environment. The IPCC (2021) reports with great confidence that global and regional climate responses to this pandemic time forcing are, however, undetectable above natural variability. While there is medium confidence that atmospheric CO2 concentrations continued to increase in 2020, there is no detectable decrease in the observed CO2 growth rate.