1 Introduction
Throughout history, man has withdrawn from the nature materials and products for their survival and life quality. Other products used by man are synthesized from materials also arising from nature. An example of this are the polymers 1. These materials are being produced in ever greater proportions and have replaced other materials in certain areas 2,3. In 1960 the polymers corresponded to less than 0.5% of urban solid waste generated in the USA and in 2009 this figure already represented 12% 4. According to Abiplast 5, in Brazil, 13.5% of the total waste generated arise from the polymeric materials segment.
The majority of synthetic polymers is produced from fossil fuel, a non- renewable natural resource, and is intended for the packaging industry 5. Due to its fast disposability, combined with its difficult degradation, plastic packages are increasing and accumulating in the environment 6. This fact is causing a growing concern with regard to environmental problems and the depletion of natural resources. In addition, this situation has led to reflection on more sustainable alternatives for production, use and disposal of polymers with the objective of minimizing the environmental damage. Among the alternatives to minimize this problem are recycling and incineration 7.
Another alternative proposed for the management of these residues are the biodegradable materials, being these more accessible to microbial assimilation, since for most synthetic polymers the degradation is a process that happens slowly and its speed depends on environmental conditions and the composition of polymers 8. The use of biodegradable polymers is an environmentally correct alternative that can replace the not biodegradable polymers, in certain applications 8.
In this scenario, the poly(3-hydroxybutyrate) PHB, is a polymer that has been causing growing interest in scientific and industrial world, because besides having thermoplastic properties and physical and mechanical characteristics similar to conventional plastics, is a biodegradable material, biocompatible and can be obtained from renewable sources 9,10,11 However, the cost of biopolymers production, as the PHB, is high and this makes them more expensive than the synthetic polymers 1,12. In Brazil, the PHB is produced by a bacterium through fermentation of sugar cane 12, which makes its cost of production economically interesting, since the sugarcane is planted in several regions 13.
The purpose of this paper is to make a literature review on the PHB, as well as on the various existing mechanisms for their production.
2 Poly(3-hydroxybutyrate)(PHB)
The poly (hydroxyalkanoate) (PHAs) constitute a group of thermoplastic polyesters, which possess properties similar to some conventional plastics from petrochemical industry 9,14, featuring thermoplastic characteristics and water resistance in relation to the synthetic polymers 15. The PHAs are biodegradable and synthesized by bacterial way, from renewable raw materials, such as, pure sugar, fatty acid or fermented liquor from waste 16. Among the PHAs the poly(3-hydroxybutyrate), P(3HB) stands out.
The poly(3-hydroxybutyrate) PHB is one of the most studied polymers occurring more frequently when the PHAs, are accumulated by microorganisms 17,18,19,20.
The literature assigns the discovery of PHB Maurice Lemoigne, who isolated it and characterized it in 1926 21. However, the date of the discovery differs among the authors of the area. Marchessault 22 mentions the period between 1923 to 1925, once that Vroman and Tighzert 23 state that this polymer began to be produced from 1925.
The PHB is an aliphatic polyester 24 with linear polymer chain 18, which presents thermoplastic properties 25. This material is nontoxic 9, optically active 26 and when pure, has a yellowish coloration 6,27, presents barrier to permeability of water and gas 6 and stability to ultra- violet radiation 28, and can be processed by extrusion, injection, blowing and thermoforming 29.
One of the most mentioned characteristics in the literature about the PHB is its biodegradability. This only occurs, however, when the material is exposed to environments biologically active 30 (soils, sea water or fresh water and aerobic and anaerobic composting), which are environments in which the material is in contact with degrading microorganisms 31. It can also be mentioned the activated sludge as another environment proper to the degradation of PHB 32 and the sanitary landfill, in which the material may be discarded without impact to the environment 6.
Another important characteristic of PHB is the biocompatibility, which can be understood as a satisfactory interaction between certain substance and the physiological environment in which is inserted. In other words, is the term used when the material is not rejected by the body and when the assimilation generates non-toxic waste. The PHB is biocompatible for two reasons: it is present in human bloodstream, in the form of PHB of low molar mass, and due to its degradation product be the acid 3- hydroxybutyric, a common metabolite in living beings 33.
The experiments conducted in the study of HUDA et al. 10 feature the PHB as a source of bioenergy, once that this material can be converted into methane gas when biodegraded. This material is also considered chemically recycable, being that the studies evidenced this recycling through experiments performed with the PHB 19,34,35.
2.1 PHB synthesis
The PHB is a material which also generates interest by presenting different forms of being synthesized 6. There are three routes of obtaining PHB, being first by means of polymerization synthetic by ring opening 23. This route uses the β-butyrolactone as reagent 23. In this process, zinc and aluminum can be used as catalysts 32 and the polymer obtained shall possess chiral center in configuration in both R, as in S, being the last named PHB racemic compound 36. Another method of obtaining is through natural plants and/or transgenic plants 11. And the third, and more widespread, strategy for the synthesis of this polymer is by means of bacterial fermentation 1,2,29,37, pure or mixed, commonly known as biosynthesis. In this process the substrate is used as a carbon source 28. It is important to emphasize that the stereoregularity of the polymer depends on your route of obtaining 23 and the stereospecificity of the enzyme responsible for the polymerization of the monomer 38. There are several species of bacteria able to accumulate the PHB and this number exceeds 70 different genera 39. These bacteria can be found in a variety of environments, in soil, water, sewer microbiologically treated 40 and even of mangrove 15. Some of the most known species of PHB bacteria are: Ralstonia eutropha 41 (nowadays Cupriavidus necator 42), Escherichia coli 9, Burkholderia cepacia and Zobellela denitrificans 15, Bacillus megaterium and Pseudomonas oleovorans 26, Protomonas extorquens, Protomonas oleovorans, Alcaligenes latus and Azobacter vinelandii 43. These bacteria can be di- vided into two groups according to the composition of the culture medium necessary for the synthesis of PHB. The first group needs, during its exponential growth phase, of an environment that is deficient in nutrients (N, Mg, S, P, K or O) and that has an excess of carbonic reserve. This situation induces the interruption of microbial cellular growth and the consequent consumption of reserve of carbon for production of PHB. The other group does not require nutritional deficiency in culture medium 26.
When metabolized by micro-organisms, the substrate is stored intercellularly as source reserve of energy 25 e carbon 44. This buildup occurs in the cytoplasm of cell in the form of granules, with diameters ranging from 0.2 µm to 0.5 µm 2.
The space occupied by granules can reach rates between 70 % to 90 % of the dry mass of the cell 38. The proposed structure for these granules is a hydrophobic core composed by PHB involved by a phospholipid monolayer. This arranges enzymes responsible for synthesis and degradation of PHB, called respectively of PHA synthase and PHA depolymerase 45. The Figure 1 illustrates what was previously described.
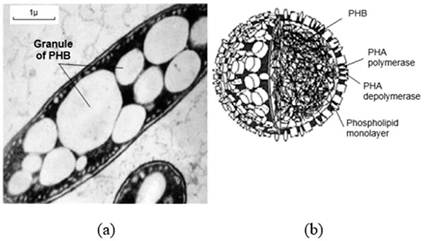
Figure 1: (a) Granules of PHB accumulated in the cell of the bacterium Azobac- ter chroococcum [2]; (b) Structure of the granule of PHB [45].
After this first stage of accumulation of PHB inside the bacterial cell (Figure 1a), the polymer is extracted and purified until reaching the shape of the final product dry and solid, when it will be ready to be processed 46. A characteristic of the polymerization of PHB that attracts world attention is that this can be operated on mild processing conditions without the minimal environmental impact 47.
The mechanism of synthesis of PHB can be described in three stages as follows 35: (a) consists in the dimming of the acetyl coenzyme A in acetoacetyl-CoA, catalyzed by enzyme β-ketothiolase (PhaA); (b) the PHA-reductase enzyme (PhaB) catalyzes the hydrogenation of acetoacetyl- CoA, forming the monomer (R)-3-hydroxy-butyryl-CoA; (c) occurs the polymerization of (R)-3-hydroxy-butyryl-CoA, catalyzed by enzyme PHB- polymerase (PhaC), forming the PHB.
The initiation of polymerization of (R)-3-hydroxy-butyryl-CoA comprehends the connection of two molecules of the same monomer the two thiols groups, belonging to the amino acids cysteine that constitute the active site of PHB-polymerase enzyme. Further it occurs the condensation of two monomers that leave one of two free thiol groups. The spread happens when a third monomer joins the thiol free group, resulting in another con- densation and so forth, generating the growth of the polymer chain of PHB 2. Figure 2 demonstrates the proposed mechanism.
In order to increase productivity, Coats, Watson and Brink-Man 48, for example, developed a study involving a microbial consortia cultured on fermented dairy manure, which under certain control conditions showed a significant reduction in PHB production costs. Similarly, Wu, Chen and Chen 49 showed that the combination of a new cell growth pattern with a morphologically altered E. coli reduced the time required for the production of PHAs, increasing the efficiency of the process of obtaining these biopolymers.
2.2 Chemical characteristics
As a member of the PHAs, the poly(3-hydroxybutyrate) is characterized by having a methyl (CH3) as alkyl replacing group 23, which confers to the material hydrophobic characteristics 6,29. In addition, this polymer is composed of monomers of 3-hydroxybutyrate that have 4 to 5 carbon atoms, which is considered a short chain lenght 26. This monomer that contains the β-hydroxybutyric acid 18, which has an alcoholic group (-OH) and a carboxylic acid (-COOH) 24. Another characteristic of the chemical structure of PHB is the presence of a chromophoric carbonyl group 28. This is illustrated in Figure 3.
2.3 Physical properties
The distribution of molar mass of a polymer is the measure of the distribution of their individual molar masses around the average molecular mass 26. In the case of PHB, this value is quite high, in range of 10,000 to 3,000,000 g/mol 26. This range of variation depends on the producer micro-organism, of carbon source and concentration, of the duration of the fermentation, the growth rate and of the polymer purifications conditions 26. The critical molar mass of this material (indicative value to which any value below this means marked loss on the mechanical properties of the polymer) is estimated at 160,000 g/mol 51.
An important characteristic of PHB is their crystallinity, because it de- fines the physical and mechanical properties of the polymer 52. The crystalline state of a material is described as being one that displays a structure set, therefore a polymer that has this characteristic is called semicrystalline.
And it is so named because its long polymer chain will never crystallize in whole as it presents a crystalline phase and another amorphous 52. The crystalline phase of PHB depends on the regularity of its structure, which in turn depends on the route that this was synthesized. O isotactic PHB 47 has chiral carbon in absolute configuration R 26,38 and is obtained by means of bacterial fermentation 23, while the syndiotactic PHB is synthesized from monomers with setting R and S track synthetic route 53. In this way, the first presents higher crystallinity than the second. In this context, the PHB presents a regular structure 54 and this will allow high crystallinity 55. Among the studies made about this material, not everyone agrees with the range of crystallinity presented by PHB. Vroman and Tighzert 23 claim that this crystallinity is above 50 %, while Sharma and Ray 56 say to be above 60 %. In both studies mentioned previously a maximum value of crystallinity was not stipulated, as what has been pro- posed by Sadi et al. 28 who declared the crystalline range of PHB as being between 50 % to 80 %. It is observed that this band of crystallinity varies widely among researchers 55 % to 80 % 57, or 60 % to 70 % 58, or 60 % to 80 % 18,59 or 60 % to 90 % 52,53.
2.4 Crystalline structure
In the crystalline phase of PHB, the molecules are arranged in an organized and repetitive way, where the smallest repetitive unit is called a unitary cell. This is composed by 4 monomeric units in a orthorhombic system, with the following dimensions: a = 5.76 angstrom, b = 12.30 angstrom and c = 5.96 angstrom [60]. The molecules have helical conformation in L (indicating swing to the left), where each complete revolution is made by two monomeric units. And present as basic forces of the model conformational, interactions of Van der Walls between the oxygen of carbonyl and methyl groups 26. This structure may be visualized in Figure 4.
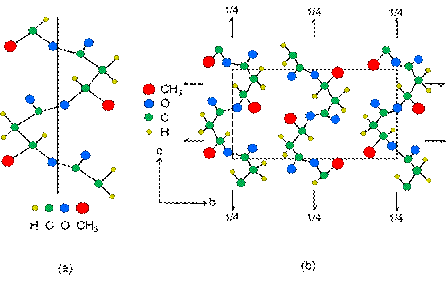
Figure 4: (a) Helical structure proposed for the molecule of PHB (the bar the axis of the polymer chain; (b) Projections of single cell of PHB in plans cb and ab 61.
2.5 Morphology of PHB
The PHB presents different structures which can be sequenced in accordance with the increasing order of its dimensions: lamellae, fibrils and spherulites. The lamellae are crystals in tape format composed by packaging in parallel of molecules of PHB, organized in a way that the axis of the polymer chain (c axis) is perpendicular to the faces of tape 62. The set of lamellae packaged in parallel is called fibrils, in which the unitary cell of PHB is arranged so that the axis "a" is oriented parallel to the radius of the spherulite 63. The union of anchoring fibrils in a central point and irradiated in all directions, with spherical shape, is called spherulites 64, that in the case of PHB are few, but large 28. Figure 5 exemplifies these structures.
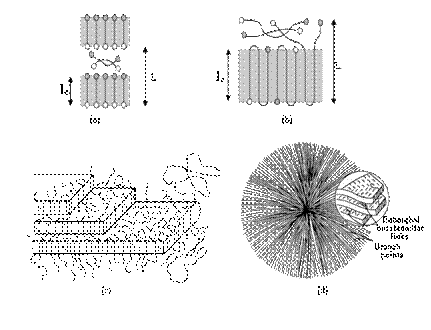
Figure 5: (a) lamellae of extended chain; (b) lamellae of folded chain 62; (c) fibrils - packaging of lamellae [65] and (d) spherulite - showing fibrils and lamellae 66.
Through an optical microscope of polarized light, the structure of the spherical spherulite can be displayed and you can still see a pattern of the Maltese cross and concentric light and dark bands, which arise by rotation of the axes "b" and "c" around the axis "a" of unitary cell 63. Figure 6 illustrates this situation.
Barham et al. 52 present a study that relates the spacing increase of bands and the radius of spherulites with the increase of temperature of crystallization of PHB. In addition, the low rate of nucleation of PHB, which generates few cores in the crystallization, makes large spherulites 51. The thickness of the lamella (lc) is equal to the length of the segment of the polymer chain crystallized in lamella, for the PHB varies from 5 nm to 20 nm 62 and this variation depends on the molar mass, of crystallization temperature and the cooling rate from the molten state 50, 61,62. When the molar mass of PHB is less than 2 X 10 −3 g.mol −1 , the widths of the lamellae will be larger or closer to the length of the extended chain, which represents that the molecules are fully extended (Figure 5a). However, when the molar mass is greater than 2 X 10 −3 g.mol −1 lamellar thicknesses will be lower than the length of the extended chain, which indi- cates that the molecules bowed to adjust to the lamellar crystals (Fig 5b) 62. The amorphous phase of PHB is located in the interlamellar spaces and intrafibrilar of the spherulites 66. The composition of this stage is of folds of chains, by segments of not crystallized string in lamellae and fraction of chain whose end is crystallized in adjacent lamellae.
2.6 Temperatures and transitions
It is interesting to know the values of some specific temperatures of poly- mers to learn how the material behaves in a temperature range, and in this way to know what the possible processabilities and applications of the same are. It is the case of the glass transition temperature (Tg), the melting temperature (Tm), temperature that initiates the reduction of molar mass, temperature of initiation of degradation and main temperature of degradation. Before starting to present the values of such temperatures, it is valid to elucidate the significance of Tg e Tm. In a semicrystalline polymer as the PHB, glass transition is the change of state from the glass state to the liquid state, in its amorphous phase. Therefore, Tg is the one in which such change happens. When temperatures are below the Tg, the segments of the chain and pending groups have restricted mobility. Now when the temperatures are above the Tg, the molecules of the polymer feature disabled translational and rotational degrees of freedom and vibrational 68.
The Tg depends on molecular parameters, such as, tacticity, molar mass, degree of crystallinity, polydispersion, branches or pending groups on the main chain [68], and experimental variables, such as, sample preparation, thermal history, rate of heating or cooling 69. It is interesting to clarify a little more about the dependence that Tg of PHB has in relation to two of these factors: the molar mass and the degree of crystallinity. Through studies observed that the Tg grows with the increase of molar mass of PHB up to 2 X 10 −3 g.mol −1 , when, from this level it remains constant 62. Now regarding the influence of the lamellar crystals in glass transition of PHB, it was observed that the higher the degree of crystallinity the higher the Tg. This is explained by the crystallization process of material that causes the confinement of segments of molecules in the interllamelar and interfibrilar spaces, which restrict translational mobility of the segments. In view of this, it is necessary the heating of the material to submit these segments to the glass transition 70. Depending on the author the Tg can have different values. Some authors suggest that the glass transition temperature is approximately 5 ◦C 6,28,57. However, values around 4 ◦C to 7 ◦C 56, or around 0 ◦C to 5 ◦C 71, or with higher temperature oscillating, in the range -15 ◦C to 9 ◦C 55, can be found.
In its turn the melting temperature is that in which the crystalline regions disappear because of the fusion of the crystallites. Due to occurring in the crystalline phase, the Tm only makes sense if applied in semicrystalline polymers as the PHB, for example. And it is in this temperature that the system power surpasses the secondary intermolecular forces of polymer chain of the crystalline phase. As a result it occurs the destruction of regular structure of packaging and the change of status from rubber for the state viscous, also called the molten state 68. The fusion of the crystalline phase of poly(3-hydroxybutyrate) is an irreversible process that can be evidenced through three ways 62,72:
The variation of the parameters Tm (melting temperature) and ∆H°m (enthalpy of fusion) with the heating rate submitted to the material;
Super cooling ∆t = T°m - Tc, indicated by the displacement of crystallization temperature (Tc) in relation to the melting temperature in the balance (T°m). When a process is reversible, both temperatures are identical;
Lamellae formed by folded chains. When a process is reversible poly- meric chains are completely unfolded in the lamellae. The thickness of the lamellae, the molar mass and the crystallization temperature are factors that directly influence in the melting temperature of the PHB. Tm increases with thicknesses of larger lamellae, with the elevation of the molar masses up to 2 X 10 −3 g.mol −1 , from this value the melting temperature varies little in a given level and with the growth of crystallization temperature, when there is the formation of thicker lamellae 62,72. The same situation of temperature variation reported in the literature, but with other values, happens with the melting temperature. Some authors 10 mention that this value is 173 ◦C, but other values can be found: 175 ◦C 6,73 or 180 ◦C 23,60. There are studies that mention ranges of variation for this temperature: 175 °C to 180 °C 56, or 170 °C to 180 °C 53, or 160 °C to 180 °C 18, ranges which cover all the values mentioned previously.
When submitted to temperatures above 170 ◦C, the PHB begins to lose molar mass 6, which leads some authors affirm that the temperature of degradation is close to this value 71. Now, in agreement with the studies of Mousavioun et al. 11, the temperature that initiates the degradation of PHB is 212 ◦C and the main temperature of degradation is 260 °C.
The melting temperature in balance (T◦m) can be conceptualized as the melting temperature of the lamellae with infinite thickness, which indicates that the polymer chains are fully extended in crystals and melting temperatures and crystallization are the same 52. T°m can vary between 179 °C to 186 °C in accordance with the molar mass, being the first value for a weighted average molar mass (Mw) of 20,000 g/mol and the second value for molar mass of 200,000 g/mol. This oscillation corresponds to the influence that the terminations of the chains exercise under the crystallization of the polymer 74.
The enthalpy (∆H°m) is usually found through the integration of the peak corresponding to the fusion process that is observed by DSC 52. The ∆H°m crystallized PHB from molten state is in function of molar mass and the history of the thermal material. However, the molar mass only influences in the enthalpy of fusion until values equal to 2 X 10 −3 g.mol −1 , for values higher than the enthalpy is practically independent of molar mass 62. For the PHB the value found for the enthalpy of fusion is of 146 J/g 25. Some of the properties described and mentioned previously, in addition to others, can be found in Table 1.
2.7 Aging and mechanical characteristics
Soon after processing the PHB goes through slow changes in the physical properties of both phases (amorphous and crystalline), causing the hardening and the weakening of the material. This process is known as the aging of PHB and its nature comes from two phenomena, the secondary crystallization and physical aging 76.
After being processed, the molten PHB begins to cool and thus it be- gins its process of crystallization, which can be divided into three parts. In the first stage, the material cools without the crystallization of its structure. Now in the second phase, the crystallization occurs in high rates, characterized as autocatalytic or crystallization. Finally, in the last stage of the process, the crystallization succeeds in low rates, reaching a stage of crystallinity self-balance, also called secondary crystallization 64.
This last step of crystallization (secondary), crystallization occurs in the amorphous phase of the polymer at ambient temperature during storage of the product 23. It is a phenomenon known by formation of imperfect crystals in the spaces of interlamellar spherulites and by the restriction of movement of the amorphous phase, which is unable to dissipate energy by viscous flow 77.
Now the physical aging can be described as the molecular relaxation that occurs due to residual mobility of polymeric chains of glass phase at temperatures below the Tg. Thus, polymeric chains of glass phase are capable of consuming changes conformational, which generates a reduction of the free volume. Therefore, the smaller the free volume, the lower the mobility of polymeric chains and that consequently brings in the hardening of the material 78.
The consequences that both phenomena carry to the physical properties of PHB are on the degree of crystallization, resistance to impact, Young’s modulus, the tenacity and elongation at break 79. The increase in the crystallinity of PHB, over time, shows that secondary crystallization occurred PHB and composites, reaching stability around 20 days with values of crystallinity varying between 43% and 47%, independently of the load presence 80. The Young’s modulus increases, indicating the rigidity of the material. In turn, the impact resistance decreases, evidencing the weakening of PHB. The value of the elongation of rupture also decreases considerably after two or more weeks of aging 79.
As already explained, the aging exerts influence on some properties of PHB as the fragility that according to Gutierrez-Wing et al. 19 decreases with the reduction of the crystallinity, the rigidity 25 and hardness 6. These characteristics grant to the PHB low mechanical properties and prevent its ability from withstanding big impacts 11. Table 2 presents some values of mechanical properties of PHB.
Table 2: Mechanical properties of PHB 81.
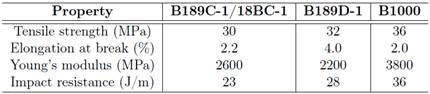
*Data provided by the manufacturer according to ASTM standards, considering different formulations; impact resistance by Izod test.
The study of crystallization and melting behavior is basic to understand and control of material properties and processing necessary to get them. An experiment was developed in order to understand the effect of the thermal cycles in the polymer phase transition suggesting that the crystallinity of PHB can be controlled by the thermal cycles of heating and cooling to which the material is subjected 82.
2.8 PHB and improvement measures
The PHB presents properties similar to some conventional plastics, as the polypropylene 83. Authors affirm that these characteristics are physical and mechanical 25. PHB also resembles polyethylene on thermo-mechanical properties 56 and with PLA in relation to mechanical properties 23. Despite of this, a difference of PHB, besides its fragile behavior, it is its thermal instability 29, being susceptible to thermal degradation when processed 55. This occurs because of their close processing window, defined by the proximity between its melting and degradation temperatures 28. In addition, this material flows during processing 6. Literature proposes several approaches for the PHB aiming to improve its mechanical behavior 25 and reduce its high cost 84. These approaches are based on the use of blends 11, obtaining copolymers 85 and insertion of additives 29.
Ke et al. 21 discuss the physical interaction of the reactive polymer blends and the formation of copolymers during the reactive polymer blending. They comment that the compatibilization can occur either by adding compatibilizers and/or the induction of chemical or physico-chemical interaction during the blending process, for example, crosslinking the compo- nents, chemically modifying the homopolymers, etc. These authors cite that the introduction of intermolecular H-bonds to polymer blends is an effective method to improve the miscibility between two components. In this case, the crystallinity and melting temperature decrease for PHB or PHBV, which is beneficial to modify the mechanical properties and en- large their processing windows. Another approach to improving properties of PHAs (linear polymers with minimal entanglement) is the formation of branching/crosslinking copolymers via reactive polymer blending. Dicumyl peroxide (DCP) possessing a relatively high hydrogen abstraction ability, makes it ideal as a branching or cross-linking agent. The same authors also discussed two other possible techniques to improve the performance of blends: formation of graft copolymers via reactive polymer blending and the formation of complex copolymers via reactive polymer blending.
Studies in the literature are found regarding the PHB/ PP blends 28,83, combination of PHB with aromatic polymers 50. Mousavioun et al. 11 points blends of PHB with poly (caprolactone) (PCL), poly (vinylidene) fluoride (PVDF), poly (vinyl alcohol) (PVOH), poly (lactic acid) (PLA), poly (vinyl acetate) (PVA), poly (vinyl phenol) (PVP), cellulose ester, and furthermore, is based on its work on blend PHB/lignin. Burlein 86 introduces in his work the blend PHB/LDPE. HO et al. 25 still mentions blends with inorganic nanoparticles, as the magnetite with size in the order of nanometers.
Bordes et al. 86 and Ke et al. 21 confirm the possibility of inserting hydroxyvalerate units (HV) between polymeric chains. It is obtained as a result of the copolymer poly(3-hydroxybutyrate-co-3-hydroxyvalerate) (PHBV), which presents lower melting point, increased this way the processing window, after the treatmentresearches of Wei, Mcdonald and STARK 87,88, PHB or PHBV were grafted with cellulose via reactive extrusion insitu DCP as the initiator. Results on surface morphology obtained by SEM revealed a better compatibility of the cellulose in the polymer matrix (PHB or PHBV) in relation to the results obtained due to the modification by means of blends. The grafting reduced the degree of crystallinity and increased the tenacity and flexibility of the biocomposites. It has been found that this simple reactive extrusion process extends the processing window for PHB-based biocomposites, which increases the range of possible applications of these biomaterials. Larsson, Markbo and Jannasch 89, showed that the termal stability of the PHAs at the processing temperature can be dramatically improved by simply washing the materials in a 1 mM aqueous HCl solution. Hence, the thermal decomposition temperature increased by up to 50 °C after the treatment. Subsequently, treated PHB and poly(3-HB-co-4-HB) were blended with different amounts of poly(butylene adipate-co-terephthalate) by melt extrusion in order to further enhance the processability and thermomechanical properties. After adding DCP during the extrusion, the interfacial adhesion improved, and the dynamic shear and tensile storage modulus increased with increasing content of the peroxide.
According Garcia-Garcia et al. 90, the compatibility of a blend PHB with PCL was improved by reactive extrusion with different DCP contents, led to a remarkable increase in ductile properties such as elongation at break and impact-absorbed energy. Addition of 1 wt % DCP also contributes to lowering the degree of cristallinity of PHB. Wei and Mcdonald 91, conducted a study blending PHB and PLLA, that were individually cross-linked with DCP (0.25 - 1 wt %) by reactive melt processing. The size of the polymer crystal spherulites, Tg, Tm and cristallinity were all de- creased as a result of cross-linking. Cross-linked polymers were also shown to be more thermally stable than linear polymers with broader molecular weight distribution (MWD) and longer chain branching (LCB) and consequente improvement in melt strength. These improvements in the properties of these materials increase your processing options, such as foaming and thermoforming.
When comparing the copolymerization products to the blend it is possible to say that this is a much faster and easier way to obtain the desired properties. Additionally, when making blends there is the possibility of incorporating cheaper polymers in relation to PHB, reducing costs and improving its mechanical properties 15,44. The introduction of additives, such as, nucleating agents, plasticizers, lubricants, antioxidants and photostabilizers, is another approach. The mixture of PHB with nucleating agents makes the average size of spherulites decrease preventing the formation of large cracks and interspherulitic on the effect of weakening 55. In addition, the plasticizers and lubricants in a blend have functions to improve molecular mobility in the molten state, decrease the Tg, reduce the termal degradation and improve the impact resistance of PHB.
2.9 Applications
Due to the characteristics of the biodegradability and biocompatibility previously submitted, the PHB presentes great potential for applications in pharmacological area 92, environmental 9, packaging 11, veterinary and industrial 17, agricultural 18 and biomedical 21,25. Some examples of applications are presented in Table 3.
It can be mentioned, as environmental benefits generated by the use of this polymer, the energy required in production processes, which is obtained by burning of sugarcane bagasse; biomass from the extraction of the polymer and effluent processes that can be applied such as fertilizers in the planting of sugarcane; and the use of solvents, natural and biodegradable, originated from the alcoholic fermentation, for purification of the polymer, all without causing negative environmental impact 30. Due to the high cost of production of PHA (generally), its commercial exploitation is significantly lower than expected The industrial scale production of PHA is limited by various factors, including the high cost of raw materials, low productivity of microbial processes (i.e., 3-4 g/(l h) PHA), the high cost of downstream processing and the separation of PHA from biomass cellular 93.
3 Conclusions
Polymeric materials have been used more and more frequently in society due to their mechanical resistance, lightness, chemical inertia and low cost of production. For this reason, these materials eventually replace metals, wood and glass in some applications. The accumulation of waste from the disposal of plastic products produced with synthetic polymers is a problem, considering the low speed of their degradation.
An ecological alternative prior to this situation is the production and use of biopolymers such as PHB. These materials are therefore classified because of the characteristic of degrade under the influence of environmental factors. PHB is a hard and brittle thermoplastic polyester with a high crystallinity index, is not soluble in water, has low thermal stability and properties that resemble polypropylene. This polymer can be obtained chemically by ring-opening polymerization and by biosynthesis. The latter is the pathway for the production of PHB best known and is performed by bacteria that synthesize and store the polymer in intracellular granules within its own cytoplasm.
Its cost of production is still high compared to other synthetic polymers, however, Brazil is one of the countries that has the lowest cost to produce PHB due to the sugarcane cultivation conducted by the alcohol industry, which is used as a substrate for its industrial production. This polymer has applications in the biomedical, pharmacological, veterinary, agricultural, environmental, industrial and mainly in the packaging sector. All these applications are possible due to two very important characteristics of PHB: biocompatibility and biodegradability.
In this review, we seek to show aspects related to the properties, the process of obtaining and the possibilities of improving the performance of the PHB. The second part of this review will discuss the different forms of degradation suffered by the PHB, at the end of its life cycle, and the necessary requirements for its occurrence.
Acknowledgements
The authors would like to acknowledge the financial support from CNPq - National Council for Scientific and Technological Development (Brazil). We would also like to thank the companies PHB Industrial S/A and Arkema Química Ltda for supplying the polymeric materials used in this research.