Introduction
MeOH is a very important raw material for the chemical industry, with an increasing demand for a wide variety of applications (Dalena etal., 2018; Hammond, Conrad, and Hermans, 2012). CH4 is one of the most important fossil fuels in the planet, which is not fully exploited because of the economical unsuitability of the synthetic pathway from syngas to produce the required quantities of MeOH (Burnett et al., 2019; Jovanovic et al., 2020). Therefore, the direct conversion of CH4 to MeOH has been a long-standing challenge in the field of catalysis (Tomkins, Ranocchiari, and van Bokhoven, 2017). Normally, MeOH is synthesized in different ways, such as CO2 hydrogenation and synthesis gas production (Abashar and Al-Rabiah, 2018; da Silva, 2016). Many efforts have been made to control the completion of the reaction up to the exact level of MeOH formation, in order to avoid overoxidation and its byproducts, e.g., carbon oxides or formic acid (Narsimhan et al., 2015; Schwarz, 2011). It is assumed that a continuous process for direct methane to methanol conversion will ultimately be limited to achieving high methanol selectivity at low methane conversions (Latimer, Kakekhani, Kulkarni, and N0rskov, 2018). Thus, the search for catalysts and reaction conditions for this process is a topical issue. A wide variety of materials have been developed as catalysts for this reaction, including copper-exchanged zeolites and mordenite (Burnett et al., 2019; Lomachenko et al., 2019; Tomkins et al., 2016; Wulfers, Teketel, Ipek, and Lobo, 2015). These kinds of materials are synthesized from different copper precursors. Zeolites with different frameworks and physical-chemical properties are used, as well as a variety of ionic exchange methods (aqueous, solid, and gaseous) (Zakaria and Ka-marudin, 2016). These kinds of materials are inspired by the activity of Particulate Methane Monooxygenase (pMMO), which is a metalloenzyme found in methanotrophs, capable of oxidizing CH4 with very high efficiency at room conditions (Banerjee, Proshlyakov, Lipscomb, and Proshlyakov, 2015; Sharma, Poelman, Marin, and Galvita, 2020). The functioning of these enzymes is given by a combination of specific copper active sites and biological-chemical processes (Balasubramanian and Rosenzweig, 2007; Yoshizawa and Shiota, 2006). Copper active sites have been broadly studied. Based on pMMO, it has been proposed that stable copper monomers, dimers, and trimmers in different configurations are able to activate methane C-H bonding due to the favourable electronic environment generated (Grundner etal., 2015; Newton, Knorpp, Sushkevich, Palagin, and van Bokhoven, 2020; Palagin, Knorpp Pinar, Ranocchiari, and van Bokhoven, 2017; Sushkevich, Palagin, and van Bokhoven, 2018). Furthermore, through various reaction mechanisms, these sites assist methane oxidation up to its specific point. Even though it has been possible to almost reproduce specific, reactive to CH4, copper active sites in the framework structure of exchanged zeolites, their yield and selectivity are still low (Jovanovic et al., 2020; Newton et al., 2020). In this context, different reaction conditions and steps within the processes have been studied to increase the quantity of MeOH produced.
Copper mordenite has been reported as one of the most efficient inorganic materials, with applications in CH4 to MeOH conversion under mild conditions (/Alvarez, Marín, and Ordonez, 2020; Burnett et al., 2019; Tomkins et al., 2016; Wulfers et al., 2015). Furthermore, it has been linked to the solid-state ion exchange synthesis method with a higher amount of MeOH production (Sainz-Vidal, Balmaseda, Lartundo-Rojas, and Reguera, 2014). Normally, experiments are focused on continuous flow reaction systems (Grundner et al., 2015; Sushkevich et al., 2018; Tomkins et al., 2017, 2019), but a static system (batch reactor) still offers a broad field to be studied, relating to the material behavior at different reaction conditions. Besides describing an experiment and predicting the results, the construction of a mathematical model allows understanding of the role played by variables, which can be modified in every reaction. It is possible to understand if and how much they are related, as well as the way in which they affect experiment performance. Herein, with the objective of elucidating the role of variables on MeOH yields, the influence of the percentage of copper charge, the temperature of activation, and methane reaction pressure in a batch process are studied using a full factorial experiment design. The choice of these variables was made according to previously reported studies, in which, starting with different copper ion exchange amounts, specific activation temperatures produce different reactive sites with various populations in the mordenite framework where the conversion process takes place. Furthermore, methane pressure is a manageable variable in batch experiments that indirectly allows the study of the interaction of a material with a gas phase, thus influencing MeOH yields (Alvarez et al., 2020; Kim et al., 2017; Tomkins et al., 2016). sectionExperimental section Ammonium mordenite with a20:1 (Silicon: Aluminium, SiO2: Al2O3) mole ratio from AlfaAesar, denoted as NH4-Mordenite, and copper (II) acetylacetonate (> 99,9%) from Sigma Aldrich as a metal ion source, denoted as Cu-(acac)2, were employed in the synthesis of materials.
Synthesis of materials: The solid-state ion-exchange method was chosen, using a planetary ball mill, to obtain homogeneous samples and control the energy applied to the samples. This is very difficult to reach if an Agate mortar and arm force are used. 0,9 grams of NH4-Mordenite with 0,0387 grams, 0,1269 grams, and 0,2334 grams of Cu-(acac)2 were grinded for 60 minutes to obtain three samples with a copper weight percentage of 1, 3, and 5, labeled as CuMor 1%, CuMor 3%, and CuMor 5%, respectively. These weight percentages were corroborated in the activated samples by inductively coupled plasma-optical emission spectrometry (ICP-OES).
Activated materials: The milled samples were dried at 70 °C for 24 hours, kept in a silica desiccator, and calcined in a muffle with airflow. Two samples (CuMor 1% and CuMor 5%) were calcined separately at 200 °C and 500 °C, thus obtaining four activated samples, labeled as CuMorO 1% 200, CuMorO 1% 500, CuMorO 5% 200 and CuMorO 5% 500. Also, CuMor 3%, calcined at 400°C (CuMorO 3% 400), was obtained.
Experimental design: A complete 2k factorial experimental design with the variables and levels shown in Table 1 was used.
Methanol obtention: Reactions were conducted in a 500 mL batch reactor (Parr Instruments, USA). 0,25 grams of each sample sieved through a < 200 pm mesh reacted with methane at the design pressure. The reactor was heated at a rate of 2 °C/min up to 200 °C and maintained at this temperature for 2 hours. After this time, it was cooled to room temperature.
Sample analysis: The resulting material was dispersed in 1 mL of water and stirred vigorously for 30 minutes to extract the formed methanol. The liquid phase was centrifuged, filtrated, and preserved in vials at 4 °C until the chromatographic analysis was carried out. Before the analysis, 1 μL of isopropyl alcohol (IOH) was added to each sample as an internal standard. The GC-MS analysis was carried out in a Clarus SQ 680 GC, coupled to a Clarus SQ 8T MS, using a PE-WAX (Perkin Elmer, Boston, MA, USA) capillary column (50 m x 0,25 mm i.d. x 0,25 μm phase thickness). The temperature program was set as follows: 40 °C for 8 min, 20 °C/min up to 80 °C, 40 °C/min up to 90 °C, and 50 °C/min up to 180 °C. The injection temperature was 150 °C, and helium was used as carrier gas (1,5 mL min-1). The mass spectrometer was operated in electron impact mode (70 EV), with selective ion monitoring (m/z 29, 31, 43, 45, 46, 58, 59, and 60; dwell time 0,05 s), keeping the chromatograph interphase and the source temperature at 280 °C. Quantitation of MeOH (mz 29+31) and IOH (m/z45) was performed using a five-point calibration curve (R > 0,99) and areas of specific ions mass-chromatograms.
Statistical Analysis: Analysis of the experimental design, general analysis of variance (ANOVA), and nonlinear regressions were performed with Statistica V 13.3 (TIBCO Software Inc., 2017).
Results and discussion
Starting with a 2k factorial experimental design, as mentioned, two central points and a random point were included. In Table 2 is summarized the experimental conditions employed and methanol yields obtained according to the experimental design described in previous section.
Table 2 Experimental design and amount of methanol obtained in moles per gram of catalyst (μmol/g) and moles per copper loaded moles (μmol Met/mol Cu)
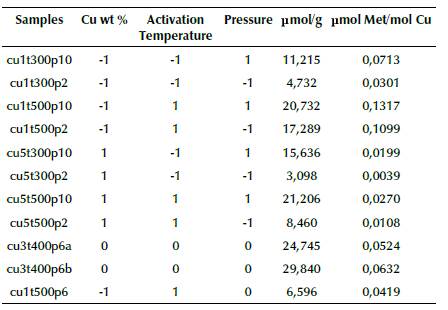
Source: Authors
It should be noted that no other compounds besides MeOH were detected in the chromatographic analysis. The obtained amount of MeOH is in the same order of magnitude with those reported in the literature (Tomkins et al., 2016, 2019; Wulfers et al., 2015), but much less compared with cycling continuous flow processes (Álvarez et al., 2020; Burnett et al., 2019; Jovanovic et al., 2020; Ma et al., 2020). Likewise, a dependency on Cu wt % of catalyst was observed (Figure 1) with a similar tendency to the work reported by Le et al. (2017) and Oord, Schmidt, and Weckhuysen (2018). Particularly, the samples with 1% and 3% Cu wt reported an increasing tendency of MeOH obtention, but samples with 5% Cu wt showed a decreased performance. This can be explained by stoichiometric calculations, in which a maximum ion exchange between 4 and 5 copper percent is evident, depending on the chemistry of mordenite and copper precursor used. More copper ions than are possible to insert into the mordenite framework tend to form different species that, at the temperatures employed in this work, did not contribute to forming MeOH (Tomkins et al., 2017). Similarly, the results in Table 2, expressed in pmol/pmol Cu, show a behavior similar to that reported in the literature, that is, lower quantities of copper loaded into the mordenite framework display a better performance in MeOH obtention (Le et al.,2017).
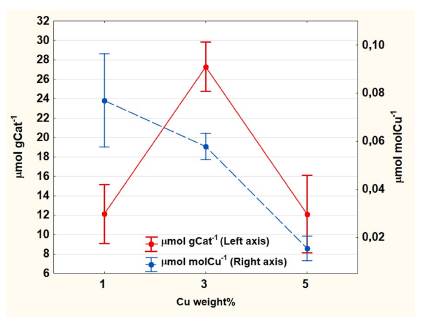
Source: Authors
Figure 1 Mean values and standard errors of MeOH obtained against copper weight percent.
The complex dependency of μmol/g yield is shown in Figure 2, in which partial minimal square adjusted surface graphics can be appreciated. It is observable that quadratic relations are needed. Certainly, the analysis of complete factorial experimental design 2 k , with a pair of central points, does not show a significative influence by any variable, nor a suitable adjustment, if the curvature (Curv) parameter is not considered in the analysis design. After eliminating the non-significative effects, a model was obtained with an intercept in which only the variables Tcal, P, and Curv were significant.
It must be noted that, at this stage, comparing the obtained and predicted data, the model does not offer sufficient certainty. At this point, a main effects ANOVA shows the global influence of variables in MeOH obtention in the studied range. The different behavior of each variable can be appreciated in Figure 3.
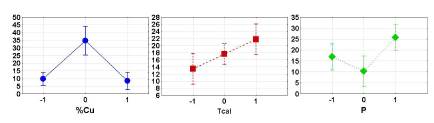
Source: Authors
Figure 3 Yield dependence with different values of variables used in the experiment, according to the experimental design (vertical bars denote 0,95 confidence intervals).
3 %Cu wt, Tcal = 500°C, and P = 10 bar are the best conditions in each of the global analysis per variables. 3 %Cu wt has a maximum yield because it contains a greater population of active sites than 1% Cu wt, which is made evident by the amount of copper ion charged in the materials. Also, it is greater than 5 %Cu wt due to a stoichiometric limitation (Dyballa et al., 2019; Pappas et al., 2017) that favors the formation of copper species which do not participate in methane oxidation. Considering the molecular shape of NH4-mordenite and the substitution of ammonium ions by copper (II) ions, the stoichiometric rate of maximum ion exchange is between 4 and 5%, depending on the mordenite's molecular formula.
Tcal = 500 °C favors the oxidation of the material, the elimination of residual organic compounds, and the production of specific active sites (Groothaert, Smeets, Sels, Jacobs, and Schoonheydt, 2005; Sheppard, Hamill, Goguet, Rooney, and Thompson, 2014). Temperatures of 300, 400, and 500 °C follow a growing trend, suggesting that higher temperatures favor the formation of a higher populations of reactive copper sites in mordenite (Sainz-Vidal et al., 2014; Vanelderen et al., 2014), associated with the amount of MeOH obtained. Activation at higher temperatures allowed us to confirm that organic material from acetylacetonate disappears, and that activation at lower temperatures does not remove it at all.
The MeOH yield has a peculiar dependence on P, showing a minimum at P = 6 atm. Pressures of 10 bar are related to a higher concentration of CH4 and greater interaction between CH4 and the material. In our experiments, a better yield was obtained at 2 bars, rather than at a 6 bar CH4 pressure. Higher CH4 conversion at lower pressures is a desired feature, and the better response at high pressures is an expected and usual aspect.
As mentioned above, the experimental model with curvature does not offer a good enough explanation to the influence of the variables on the MeOH yield. Therefore, a polynomial regression was employed with the coded values of the variables, in which the quadratic influence of %Cu and P was observed with a good description of results. The quadratic term for Tcal was zeroed with the statistical package. Figure 4 shows how far away the experimental data are from those predicted by the proposed model.
Finally, to verify the goodness of the quadratic model, and to obtain the model coefficients based on the real values of the variables, a nonlinear estimation was performed using Equation 1, considering that the yield has a quadratic dependence on %Cu and P, and a linear dependence on Tcal, as it was obtained with the quadratic regression. It was observed, as expected, that residuals are like those obtained with the previously discussed model. The coefficients A, B, C, L, Q, and R in Table 3 were obtained using the Levenberg-Marquardt estimation method of the statistical package.
All terms were considered because the entire p-values of the coefficients were under 0,05. In Table 3, the estimate values of coefficients according to the nonlinear model, which are represented in Equation 1, can also be observed.
This nonlinear model allows a good description of the experimental points in terms of the real values of the studied variables.
Conclusions
A nonlinear statistical model of a batch reaction of methane to methanol direct conversion employing copper mordenite as the catalyst is proposed, which describes with good accuracy the experimental results of the yield of methanol production. The model adequately confirms what is seen in Figures 1 and 3, that is, very high or very low values of a copper-loaded percent can lead to a decrease in the yield, which is a fact that has been observed in practice. The maximum value at 3% Cu wt can be explained according to the formation process of copper mordenite, with the maximum stoichiometric copper percent ranging between 4 and 5%, depending on the exact mordenite molecular framework unity and precursor of copper employed. Higher amounts of Copper-loaded into mordenite (> 5% Cu wt) may have a negative effect on methanol production with the conditions applied in the experiments. This suggests the important role of the catalyst stoichiometry in the yield of methanol production. On the other hand, pressure behaved as expected, and higher pressure means bigger concentration and contact between methane and material and, consequently, a major probability of interaction of methane with the mordenite's active sites. At lower pressures, the interaction is reduced and, as a result, decreases the amount of methanol produced. However, the material still produces good enough quantities, suggesting that pressure does not have such a great impact in methanol production within the range studied. Within global analysis, the mordenite's calcination temperature had a linear behavior at higher temperatures, and a higher yield of methanol was obtained. However, in relation to the copper-loaded amount, this variable seems to have a low influence on the experimental results, as is suggested by its linear contribution to the model. To the best of our knowledge, a nonlinear model that describes the yields of the batch direct conversion of methane to methanol has not been proposed, which gives practical value to the results herein obtained from an engineering point of view. More experimental data are needed to improve the accuracy of the model and to expand the studied variable ranges.